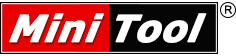
- Disk & Data Manager
- Partition Wizard
- Power Data Recovery
- ShadowMaker
- Media Toolkit
- uTube Downloader
- Video Converter
- Download Partition Wizard Free Edition: Download Pro Edition: Try Demo Server Edition: Try Demo

5 Solutions to Processor Thermal Trip Error on Windows 10/11
To avoid data loss due to unexpected errors like processor thermal trip error, you’d better make a Windows backup via PC cloning software like MiniTool Partition Wizard. It helps you clone the whole system disk with ease. Besides, this multifunctional partition manager also allows you to convert MBR to GPT , migrate Windows 10, wipe disks, recover missing partitions/data, etc.
MiniTool Partition Wizard Demo Click to Download 100% Clean & Safe
If you are suffering from processor thermal trip error on Windows 10, try the methods below to get it solved.
- Check the ventilation fans
- Check thermal paste and heat sink
- Disable overclocking
- Disable background processes
- Increase RAM
Solution 1: Check the Ventilation Fans
When the processor thermal trip error occurs, the first thing you should do is check if the ventilation fans are overheating. If it gets overheating, cool down by taking some measures. For instance, remove any obstructions near the fans to get enough airflow.
Wipe the dust off the ventilation fans to make sure that the fans work properly. Besides, put the computer in a place that is not too hot or humid.
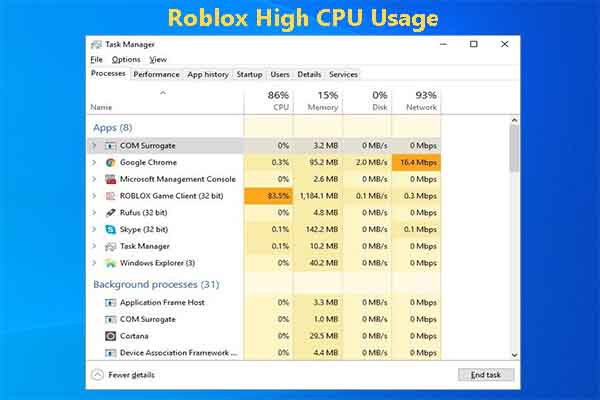
How to fix Roblox high CPU usage? You may raise this question like others. If you are suffering from it, apply the given methods to solve it.
Solution 2: Check Thermal Paste and Heat Sink
Insufficient thermal paste between the CPU and its heat sink may trigger the processor thermal trip error in Windows 10/11 too. The thermal paste acts as a cooling agent, which can drastically cool down your system through the fan.
However, if the thermal paste is not applied properly, it won’t do its job and then your processor will get overheating. Hence, it is vital to check the thermal paste and heat sink on your computer. To do that, please follow the steps below.
- Turn off the computer and then remove the fan on the top of the processor by unscrewing the bolts with care.
- Apply the thermal paste on the top of the processor.
- Screw the fan again in its place.
- After some time, reboot the PC.
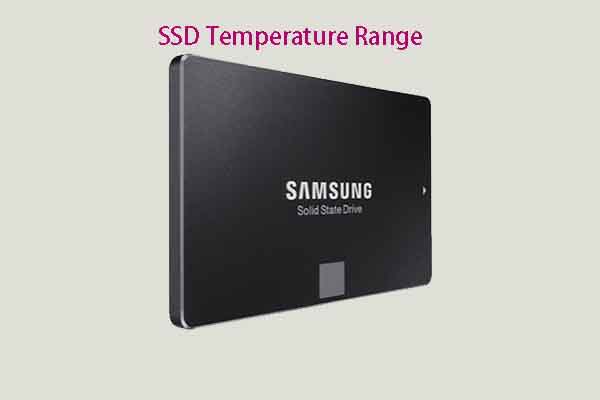
What’s your SSD temperature range? If you aren’t clear about it, you can check that by referring to this tutorial.
Solution 3: Disable Overclocking
Overclocking boosts the processor clock rate for a short time period, but it also increases the temperature sharply. If overclocking doesn’t stop in time, the processor thermal trip error will occur. So, disable overclocking after you meet this error. Here’s the step-by-step guide.
Step 1: Open the Settings app and then click Update & Security .
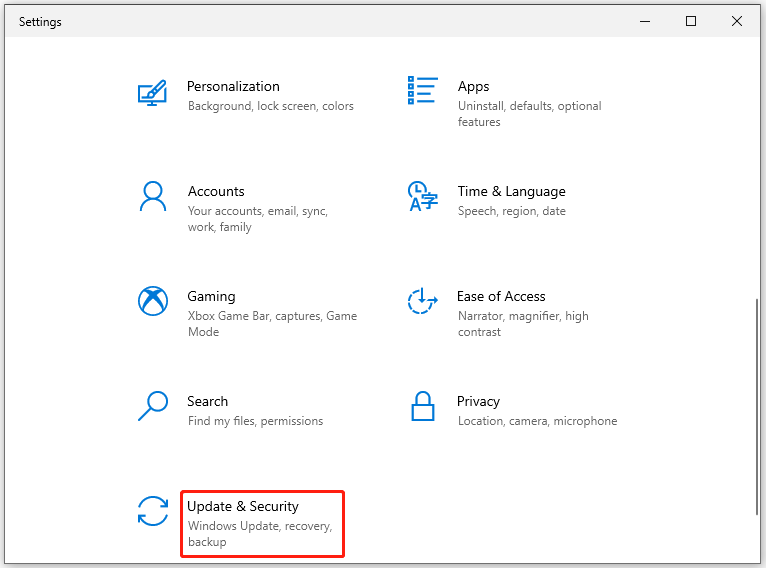
Step 2: Tap on Recovery in the left panel.
Step 3: Then click on the Restart now button on the right side of the window.
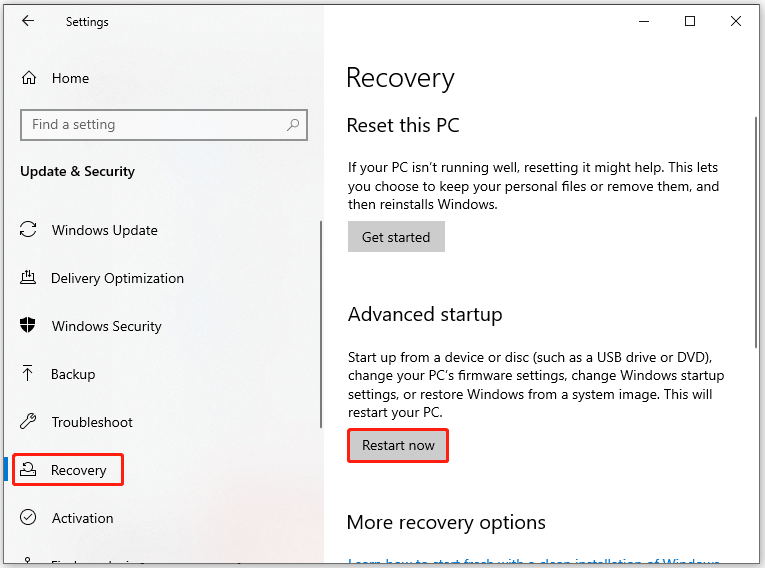
Step 4: After your computer restarts, click Troubleshoot > Advanced options > UEFI Firmware Settings > Restart .
Step 5: Then your computer will restart and open BIOS automatically.
Step 6: Click on the Advanced tab in it and then tap Performance and choose Overclocking .
Step 7: Now, disable the Overclocking option.
Step 8: Press the F10 and Enter keys to confirm and execute the changes.
Also read: 2 Simple Methods to Enable or Disable Dev Drive Protection
Solution 4: Disable Background Processes
Step 1: Right-click on the Windows icon to open the Start menu. Then click Task Manager from the menu.
Step 2: In the Task Manager window, tap on the Processes tab and then find the processes that have high CPU usage.
Step 3: Right-click on the process with high CPU usage and then click End task . Repeat this operation to close all CPU-consuming processes.
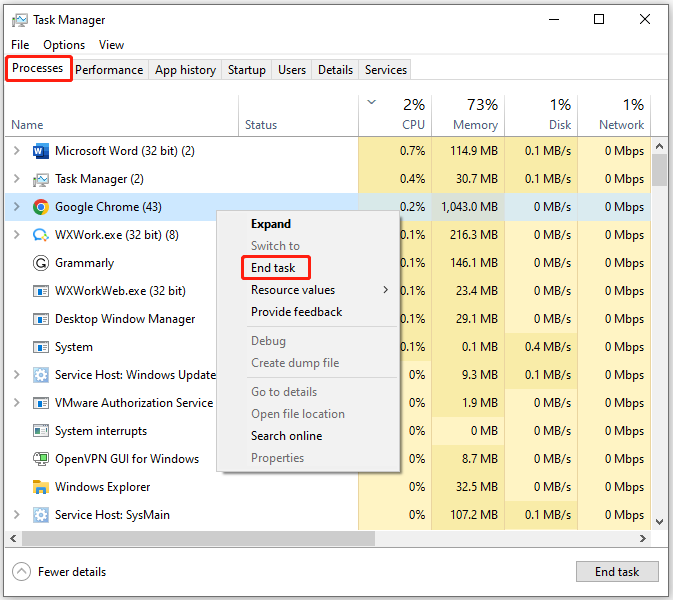
How to enable or disable Windows Recovery Environment? If you are seeking methods, this post deserves your attention as it collects 2 ways.
Solution 5: Increase RAM
If RAM on the computer is not enough and requires more space due to less memory, it can cause the system to slow down, freeze, or overheat. Then you may receive the processor thermal trip error on Windows 10. To get rid of the processor thermal trip error in Windows 10/11, you need to increase RAM on your computer. If you don’t know how to do that, simply follow this tutorial: How to Get More RAM on Laptop – Free up RAM or Upgrade RAM
About The Author
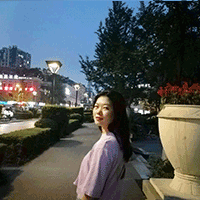
Position: Columnist
User Comments :
Techs & Gizmos
Hands On Review For Latest Gadgets & Gizmos
How to Fix Thermal trip error Windows 10/11 [Steps]
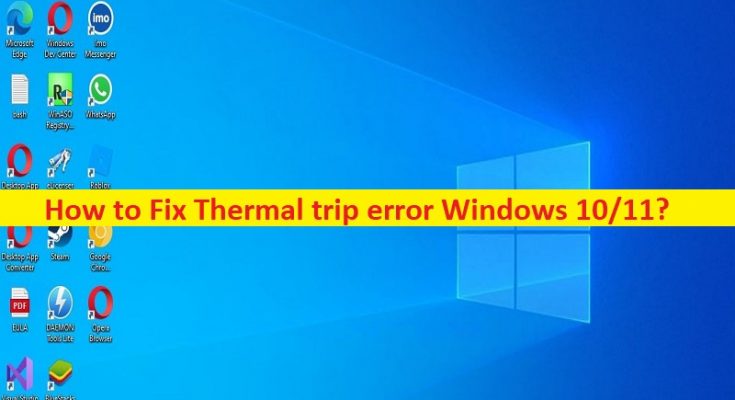
What is ‘Thermal Trip error’ in Windows 10/11?
In this article, we are going to discuss on How to fix Thermal Trip error Windows 10/11 . You are provided with easy steps/methods to resolve the issue. Let’s starts the discussion.
‘Thermal Trip error’ – Processor Thermal Trip error:
It is common Windows PC Problem considered as Processor Thermal Trip error. The Thermal Trip prevents the CPU from overloading and roasting. It is cooling system that is dedicated to making sure that the processor of system is cool at all times. In case the CPU gets hot, the system turned OFF, and if the system has crossed the cooling threshold and has become too hot, you might face Thermal Trip error on Windows computer. This issue prevents you from using your Windows computer comfortably.
The possible reasons behind the issue can be the interference of background processes, issue with overclocking, insufficient RAM or memory, and other issues. You can try to fix the issue by disabling overclocking CPU/GPU in your device in order to fix the issue. It is possible to resolve the issue with our instructions. Let’s go for the solution.
How to fix Thermal Trip error Windows 10/11?
Method 1: clean dust from cpu’s fan and other hardware components.
You can clean dust from CPU’s fan and other hardware components like RAM or memory in order to fix the issue. To do so, you need to shutdown your computer and open CPU case, clean dust from CPU’s fan, memory, RAM and other CPU cabinet portion using a soft cloth and then close the CPU case, and then turn ON your computer and check if the issue is resolved.
Method 2: Uninstall recently installed software
You can uninstall recently installed software or problematic software in computer in order to fix the issue.
Step 1: Open ‘Settings’ app in Windows PC and go to ‘Apps > Apps & Features’
Step 2: Find and select the recently installed apps or problematic apps, and select ‘Uninstall’ to uninstall it. Once done, restart your computer and check if the issue is resolved.
Method 3: Close background process
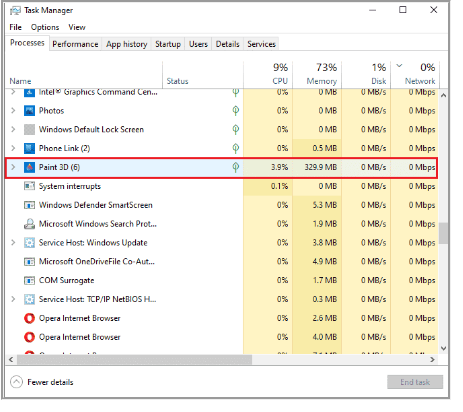
Step 1: Press ‘CTRL + SHIFT + ESC’ keys on keyboard to open ‘Task Manager’ app
Step 2: Right-click on process that is consuming the CPU resources, and select ‘End Task’ to kill them and once done, check if the issue is resolved.
Method 4: Disable overclocking
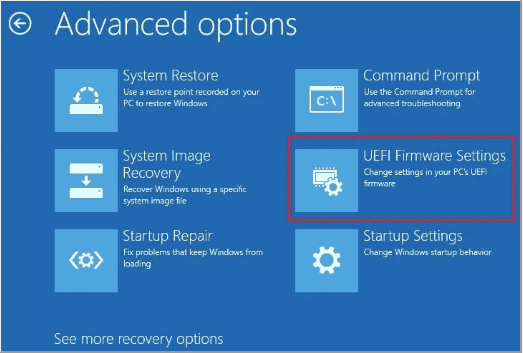
Step 1: Open ‘Settings’ app in Windows PC and go to ‘Update & Security > Recovery’ and click ‘Restart Now’
Step 2: Select ‘Troubleshoot > Advanced Options > UEFI Firmware Settings’ and select ‘Restart’
Step 3: Now, go to ‘Advanced’ tab and click ‘Performance > Overclocking’, and disable the overclocking option
Step 4: Once done, save the changes and exit BIOS, and restart your computer and check if the issue is resolved.
Method 5: Increase RAM
This issue can be occurred due to insufficient RAM or memory space in computer. You can increase RAM from 2GB to GB in order to fix the issue, and then check if it works for you.
Method 6: Check core temperature
You can check core temperature of your system using a third-party software in order find and fix the issue.
Step 1: Download Core Temp from its official site or visit ‘https://www.alcpu.com/CoreTemp/’ page
Step 2: Once installed, launch the application and check the temperature in front of each one.
Step 3: If the core temperature of system is above the standard temperature that is between 40 Degree Celsius and 65 Degree Celsius and none of fixes have worked for you, then you can get your system check by a hardware expert.
I hope this post helped you on How to fix Thermal Trip error Windows 10/11 with easy ways. You can read & follow our instructions to do so. That’s all. For any suggestions or queries, please write on comment box below.
Related Posts
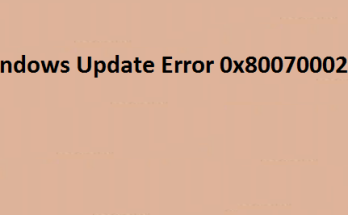
How to fix Windows Update Error 0x80070002? Simple and Easy Methods
September 12, 2024
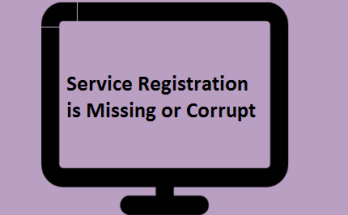
How to fix Service Registration is Missing or Corrupt Error?
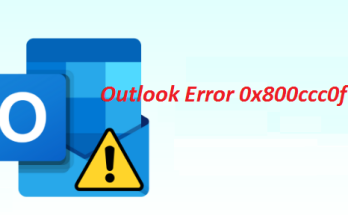
How to fix Outlook Error 0x800ccc0f?
September 11, 2024
About Sachin Prakash
Privacy overview.
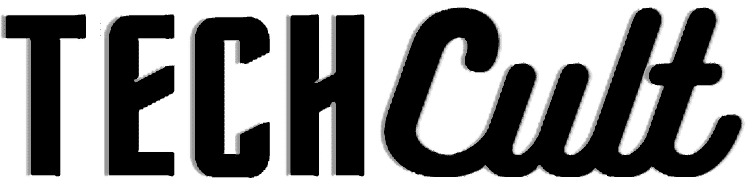
Fix Thermal Trip Error in Windows 10
A thermal trip prevents the CPU from overloading and roasting. It is a cooling system that is dedicated to making sure that the processor of the system is cool at all times. In case the processor gets hot, the system shuts down. If your system has crossed the cooling threshold and has become too hot, your system might be facing a thermal trip error. If you are someone looking for solutions to this problem then you are in the right place. Not only will we educate you about solutions for the issue but we will also acquaint you with what causes thermal trip. So, let us get into detail about the issues causing your system to heat and suitable fixes for them.
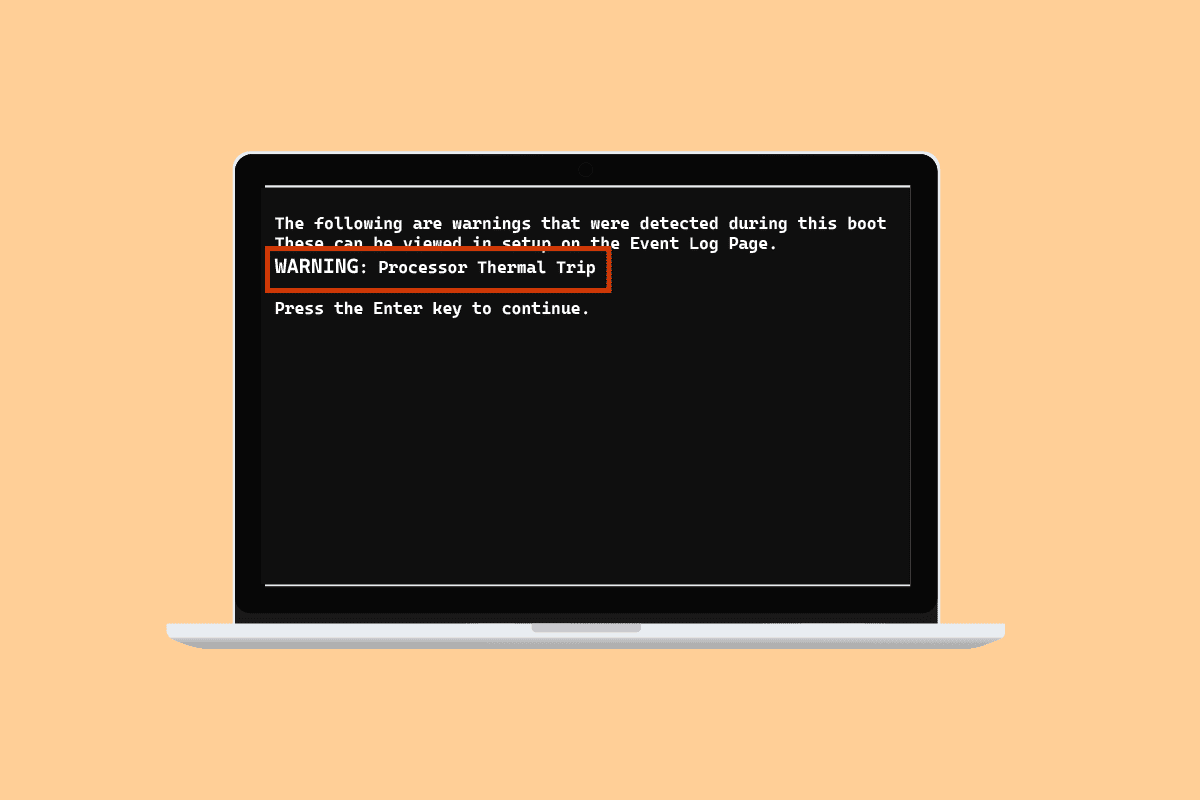
Table of Contents
How to Fix Thermal Trip Error in Windows 10
The dominant and most common reason that has been seen to cause thermal trip error in Windows 10 is a failed cooling system of the process. Apart from it, what causes thermal trip has been explained in the points given below:
- An unclean computer can cause the cooling system to get blocked by dust which leads to the error.
- Another reason behind the Thermal trip can be overclocking which along with increasing clock rate also elevates the temperature.
- If the processor is not installed properly, it can also be the reason behind Thermal trip issue.
- If an application or a game on your system does not have sufficient resources, it can cause an error.
- A virus or malware in the system can also trigger thermal trip problems.
Usually, the methods used to fix Thermal trip issue are physical methods like cleaning the computer, checking the fan, and more. As basic as these solutions may seem, they are equally critical in helping you out with the problem. So, let us begin by knowing more about these troubleshooting methods first:
Method 1: Basic Troubleshooting Methods
Try the following basic and simple steps before you move to more advanced methods:
1A. Check Ventilation Fans
The first and foremost thing that needs to be checked in case of overheating is the fan. Also, you need to check if there are any obstructions near the vent that are blocking its way from performing the cooling function. If you are using a laptop, you can make sure that its airflow is not obstructed by keeping it on your lap rather than on a desk.
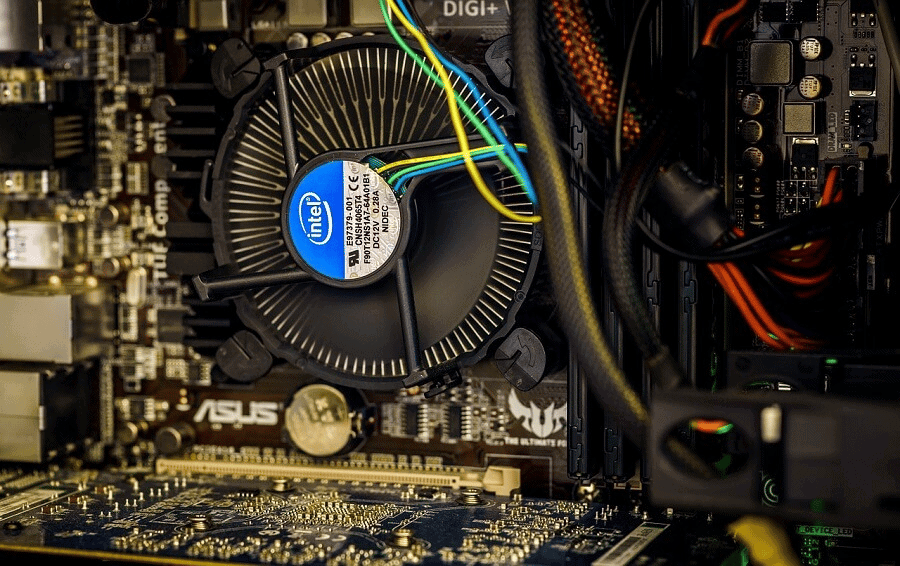
1B. Check the Environment
Another reason that is usually untouched is the concern of the environment in which the system is working. Too hot or humid environments have also been seen to affect how systems respond. Hence, if you are working in an overheated circumstance; it can lead to thermal trip error.
1C. Run Malware Scan
The next troubleshooting method to apply as a solution for Thermal trip fix is to run an anti-virus scan or a malware scan on your system as a virus attack has also been seen to cause the issue. You can check our guide on How do I Run a Virus Scan on my Computer .
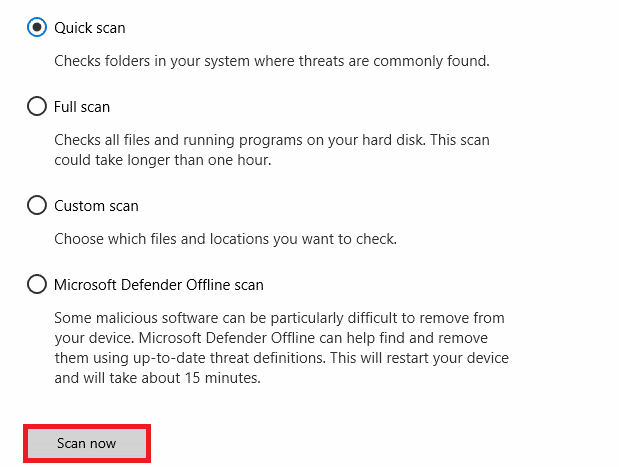
Also Read: Fix Ntoskrnl.exe High CPU Usage
1D. Uninstall Programs
If you recently have installed a program or an application on your system and since then have started to encounter the thermal trip issue then the installed program may be behind the error. To rule out this possibility, you must uninstall the program using the following steps:
1. Right-click on the Windows icon on the screen, type Apps and Features & click Open as shown.
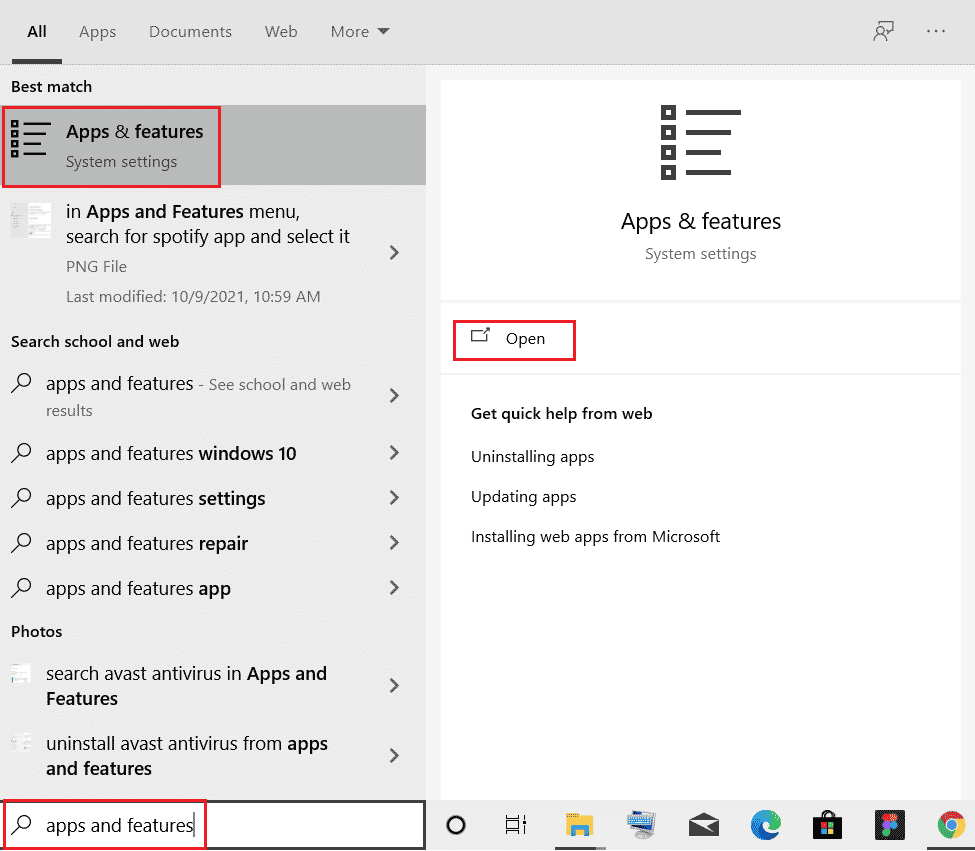
2. Locate the program (e.g. CCleaner ) with the help of the search list and click on it.
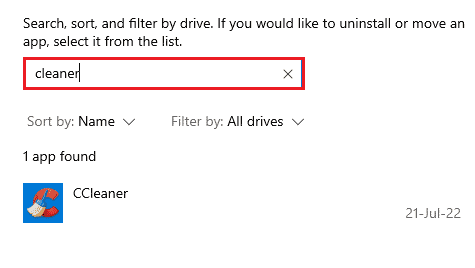
3. Select Uninstall .
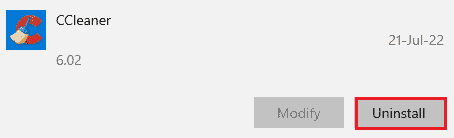
4. Click on Uninstall to confirm.

Method 2: Close Background Processes
The next thermal trip in laptop solutions list is to check the CPU usage of the system. The fan will run faster and the system will put more strain on the resources if certain programs or processes put more strain or take up more disk space. If this is the case then you can check the issues mentioned using the Task Manager.
1. Hit the Ctrl + Shift + Esc keys simultaneously to open Task Manager .
2. Check the running processes under the Processes tab that are causing high CPU usage.
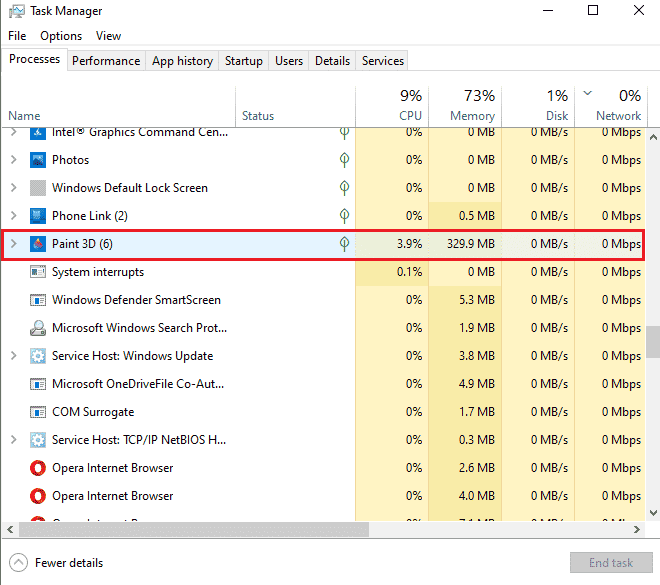
3. Right-click on such a process (e.g. Paint 3D ) and select End task .
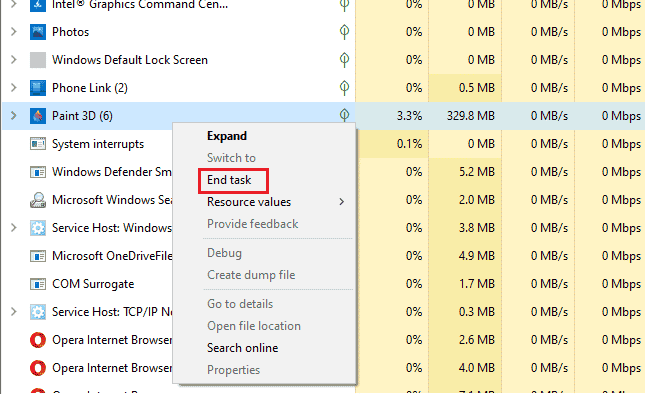
If the above-mentioned steps did not help you with the CPU usage issue, then you can read our guide on How to Fix High CPU Usage on Windows 10 for more.
Also Read: Fix Laptop Thermal Throttling
Method 3: Disable Overclocking
The next solution that we have in front of us is related to overclocking. As discussed in the causes above, overclocking results in overheating, and eventually, it causes the error. For that reason, disabling overclocking is crucial to get over Thermal trip error and cool down your system. Following are the steps that can help you with disabling it:
1. Hit the Windows + I keys together to open Settings .
2. Then, click on Update & Security in it.

3. Select Recovery from the left pane.
4. Click on Restart now.
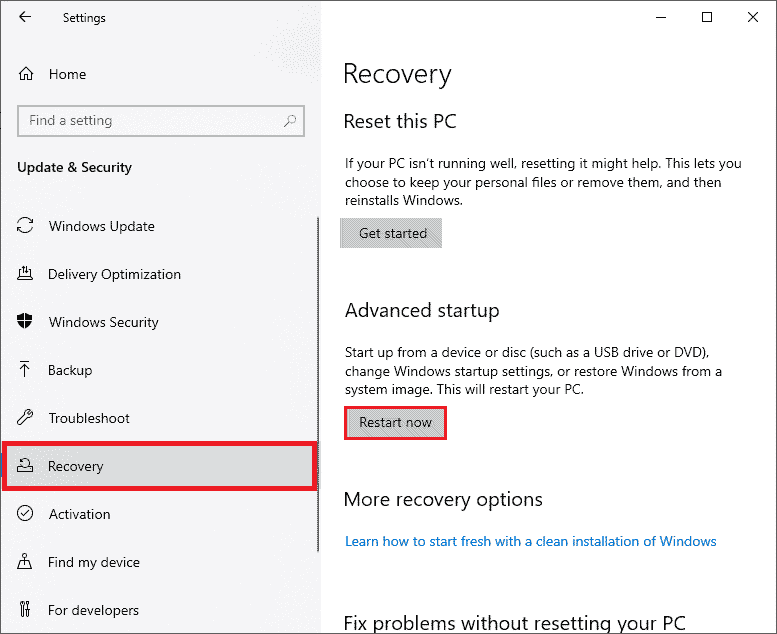
5. When the system restarts, select Troubleshoot .
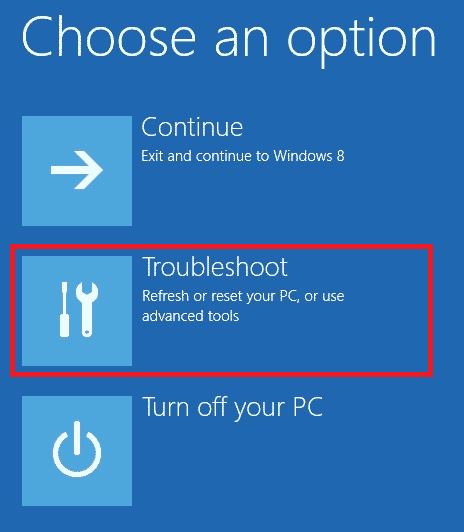
6. Next, select Advanced options .
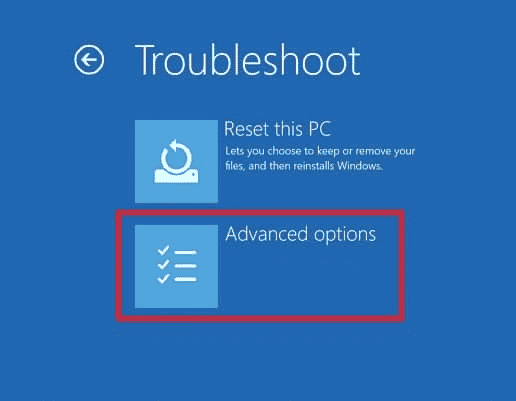
7. Now, click on UEFI Firmware Settings and select Restart .

8. Once the system is rebooted, it will open BIOS automatically, select the Advanced tab in it.
9. Then, click on Performance and select Overclocking .
10. Now, disable the overclocking option .
11. At last, press the f10 key to save the changes to BIOS and then start the system normally.
This is one of the effective Thermal trip in laptop solutions.
Method 4: Increase RAM
The next method that can be helpful in Thermal trip error fix is to increase the RAM. It is possible that RAM in your system is less than sufficient and requires more space due to less memory, this can trigger the system to slow down, freeze, or cause overheating and therefore the error. So, in such a case increasing the RAM from 2 GB to 4 GB can help you out. You can also check our guide How to Check RAM Frequency on Windows 10 for more information about RAM on your system.
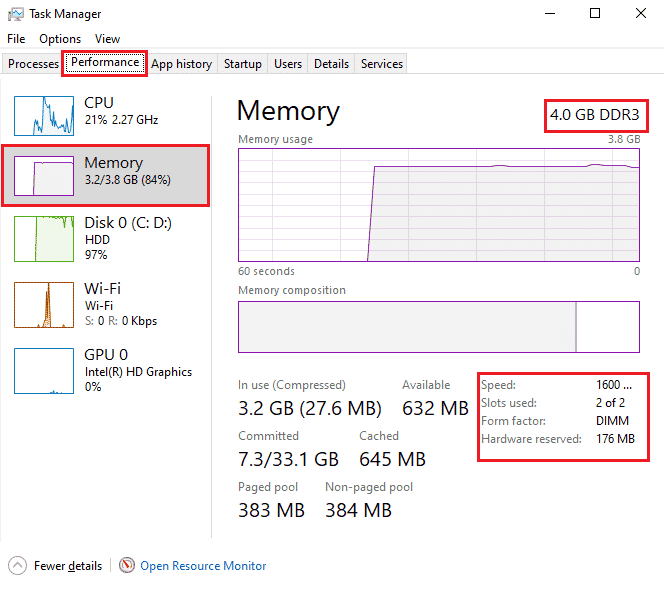
Also Read: How to Check Your CPU Temperature in Windows 10
Method 5: Check Core Temperature
If none of the methods has helped you in resolving Thermal trip error issue then you can try checking the core temperature of your system using a third-party software program. With its help, you will be able to compare the temperature of your system with the standard core temperature.
1. Open Core Temp’s official site and download the software.
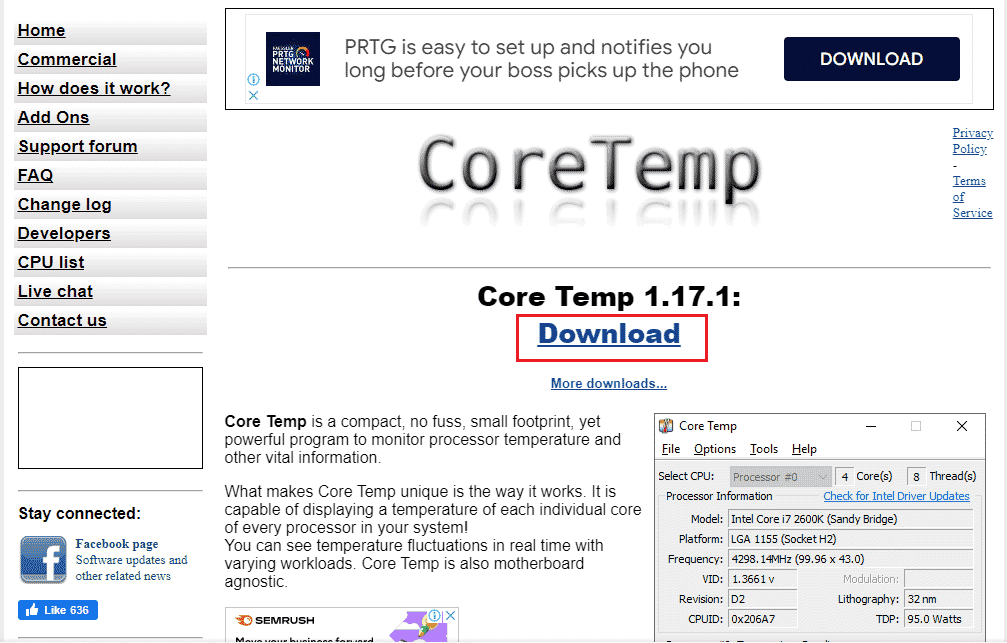
2. Once installed, launch the application and check the temperature in front of each core.
3. If the core temperature of your system is above the standard temperature that is between 40 Degree Celsius and 65 Degree Celsius (or 104 Degree Fahrenheit and 149 Degree Fahrenheit ) and none of the fixes have worked for you then you can get your system checked by a hardware expert.
Pro Tip: Check Thermal Paste and Heat Sink
The Thermal paste helps in drastically cooling down your system through the fan. If the ventilation system is working alright for your device and you are still facing Thermal trip in laptop solutions error then it is plausible that the thermal paste on your process is not correctly applied. In this case, the processor can get overheated and result in the error. Therefore, it is important to check the thermal paste and heat sink on your processor:
1. Remove the fan by carefully unscrewing the bolts just right above your processor .
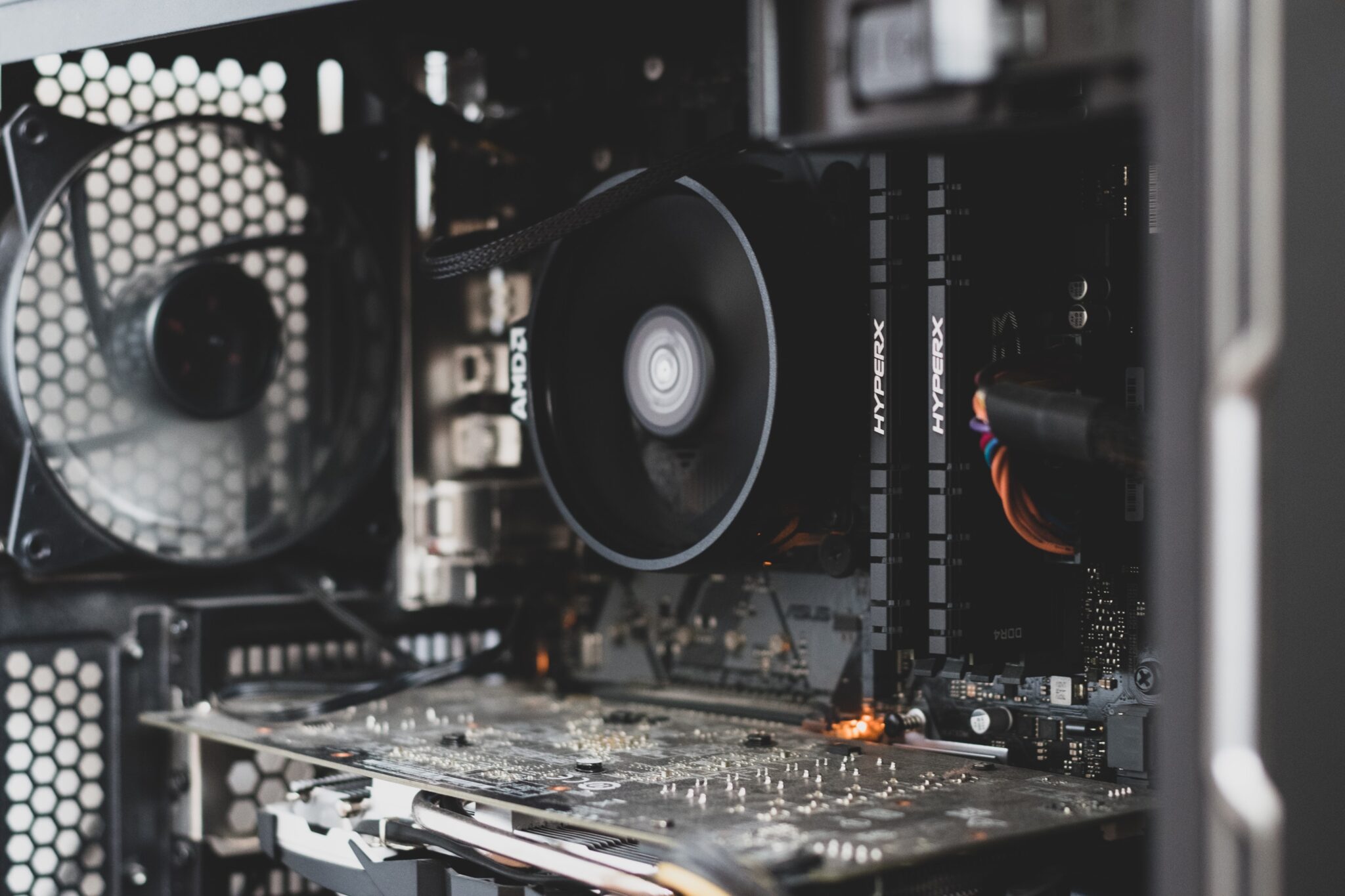
2. Apply the cooling agent on top of the processor.
3. Now, screw the fan again in its place.
4. After some time reboot the PC .
Hence, these are Thermal trip in laptop solutions.
Also Read: 7 Ways to Fix CPU Fan Not Spinning
Frequently Asked Questions (FAQs)
Q1. What temperature is dangerous for a computer?
Ans. If your system runs under 65 Degree Celsius , there is nothing to worry about. But anything that crosses the 70 Degree Celsius mark is dangerous for your system and it needs to be cooled down.
Q2. What is a thermal shutdown in a PC?
Ans. A thermal shutdown in a PC happens when the processor or CPU’s temperature reaches a critical level which results in an automatic shutdown of the system to avoid any permanent damage.
Q3. What is meant by thermal trip?
Ans. A thermal trip is a bimetal thermal device that protects against overloading in a system. It results in the opening of a circuit breaker with a delay.
Q4. If my computer overheats for a long time, what will happen?
Ans. System overheating for a longer period results in your computer being unstable and eventually shutting down . It can also result in potential component damage .
Q5. How long does the thermal paste last?
Ans. Thermal pastes last for about 2-3 years in the case of normal compounds. While for higher-end compounds the limit is 7 years .
Recommended:
- How to Find Your Lost Phone Using Lookout
- Fix WASD and Arrow Keys Switched in Windows 10
- What is Clock Interrupt?
- Fix System Error 5 Access Denied in Windows 10
We hope that our guide helped you in all ways possible to fix Thermal trip error and to know about what causes thermal trip. Let us know which one of the methods guided you the most in resolving the issue. If you have any more queries or have some valuable suggestions to give then drop down a comment for us.
About The Author
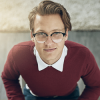
Elon Decker
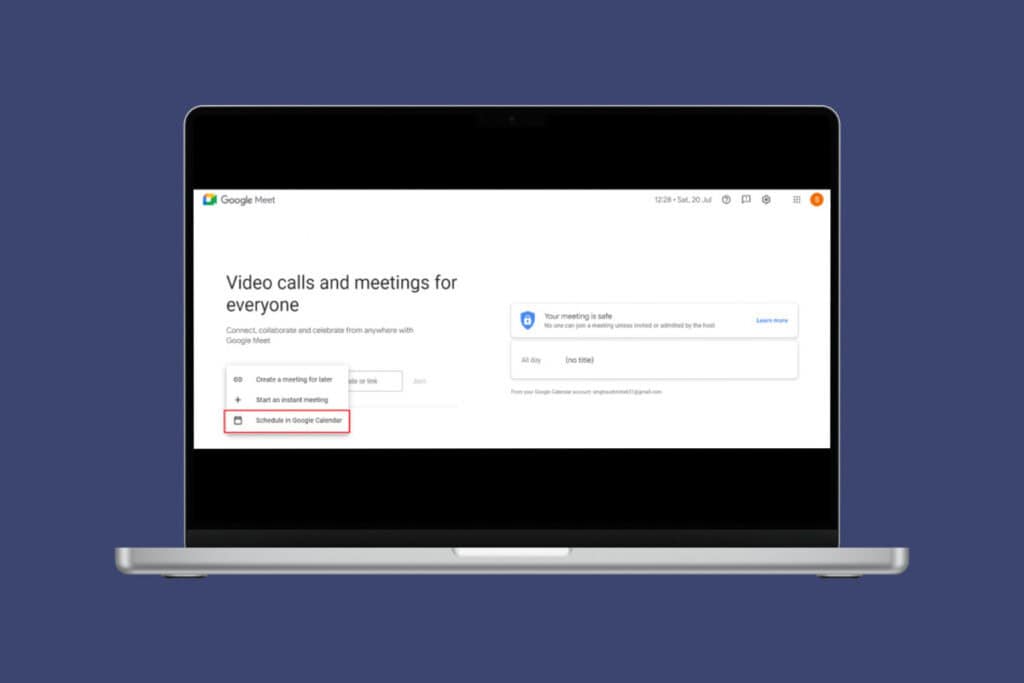
How to Schedule a Meeting on Google Meet
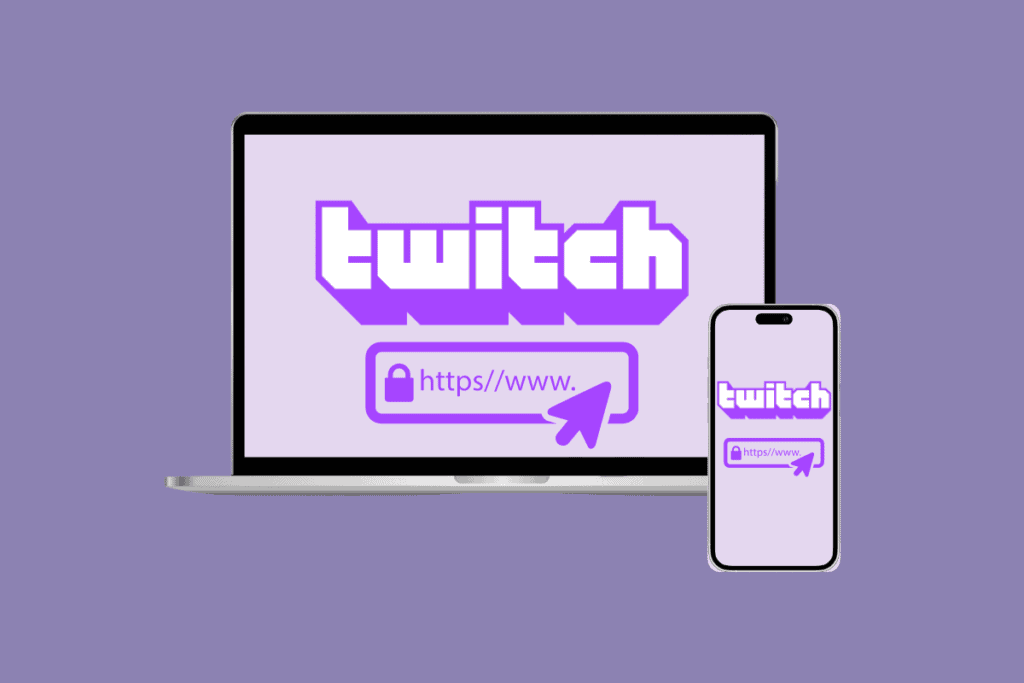
How to Copy and Share Twitch Link on Windows and Android
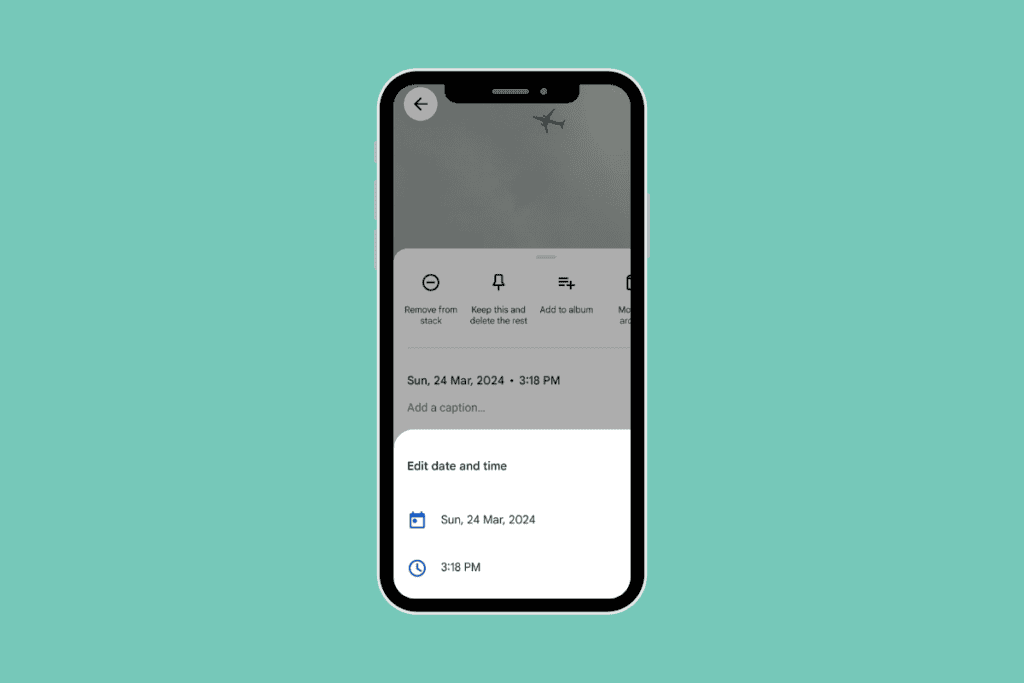
How to Change Date and Time of a Photo on Android
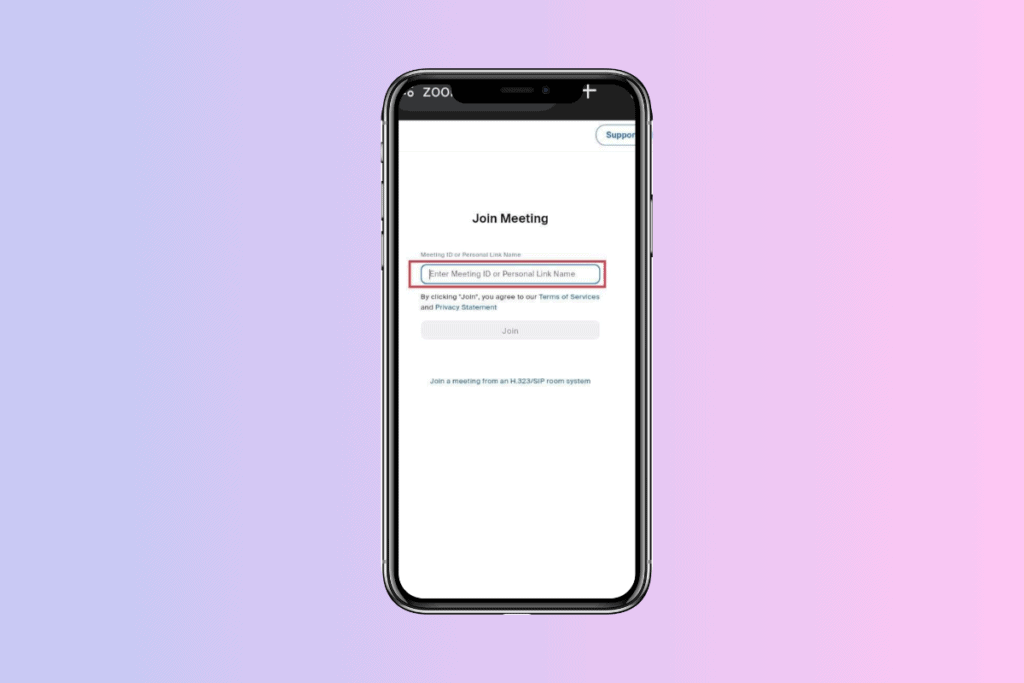
How to Use Zoom on Android Phone Without App
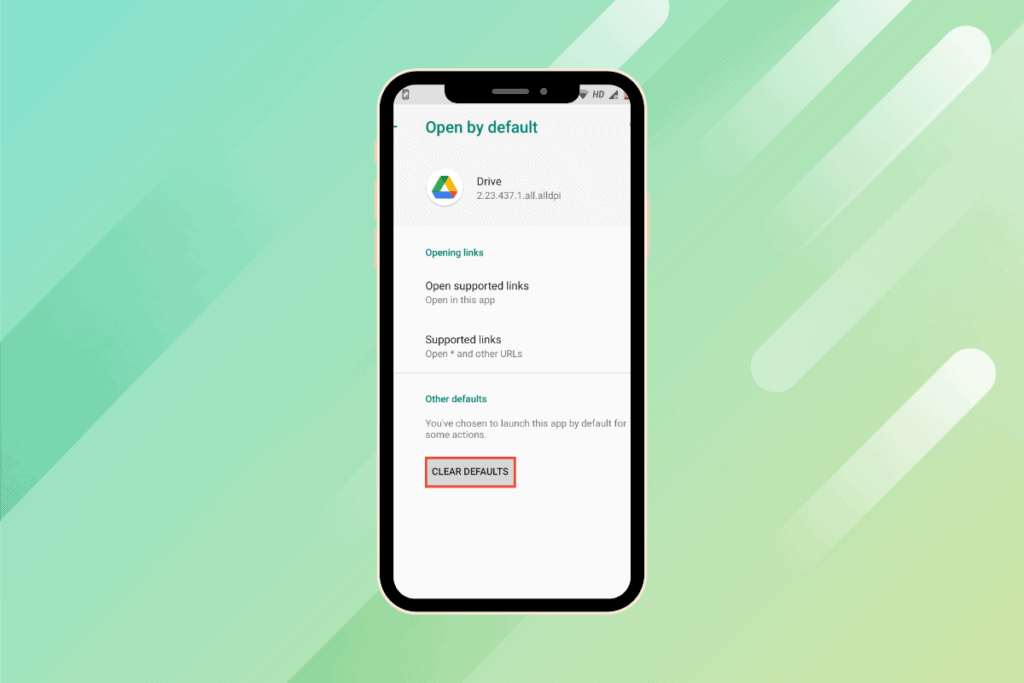
How to Change Default PDF Viewer on Android
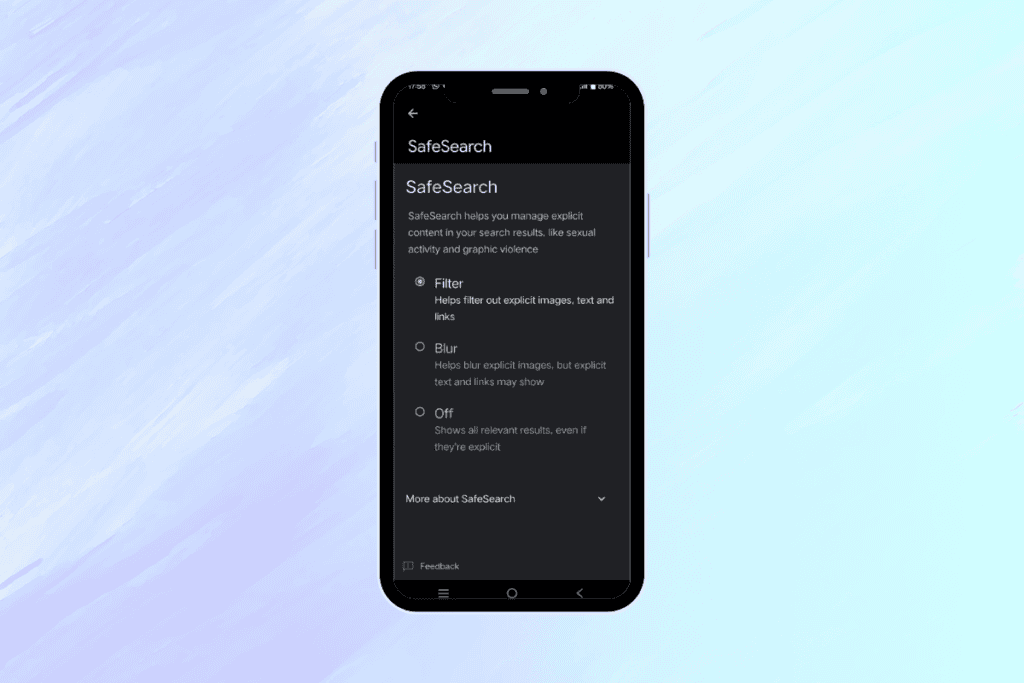
How to Restrict Google Search on Android
Leave a comment cancel reply.
Your email address will not be published. Required fields are marked *
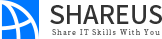
- >
Processor Thermal Trip Error in Windows 10/11: Here’s how to fix it

September 1, 2022
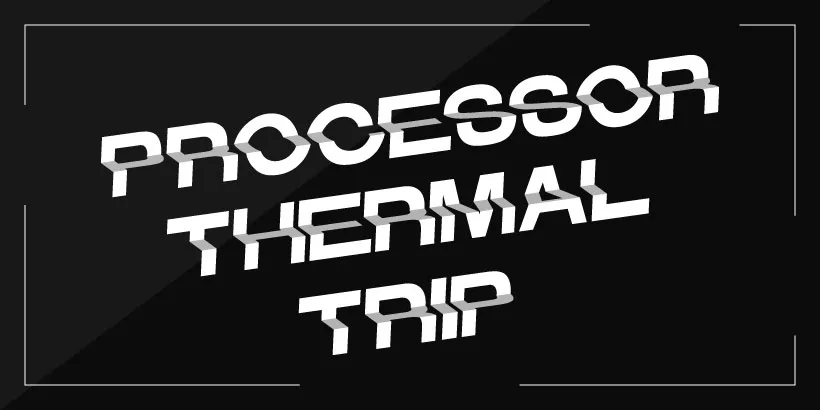
Typically, a CPU is configured to perform normally within a specific temperature and once its temperature exceeds a safe threshold, the thermal trip is activated. The processor thermal trip is meant to protect the CPU from being overloaded by continuous intense tasking, shutting down the system when the processor overheats, thus ensuring that the processor remains at a cool temperature. You may get the "Thermal trip failure" error message after a forced shutdown, which implies the temperature of your processor is reaching its threshold. In this post, we will discuss why could this issue happen and what to do if thermal trip error appears .
Why processor thermal trip failure would happen?
How to solve the processor thermal trip error, 1. check core temperature, 2. check the ventilation fans, 3. check thermal paste and heat sink, 4. close background process of high cpu usage, 5. disable overclocking, 6. increase ram.
- Insufficient thermal paste between the processor and heat sink or the heat sink is not securely fastened.
- Fans are not working properly or too much dust is accumulating around the vents.
- Overclocking leads to overheated processor.
- CPU-intensive applications burdened the system.
Before we performing the following fixes, it is important to note that do not turn on the computer immediately after a thermal shutdown. You will have to wait until the components inside the computer have cooled down completely. The cooling time depends on the room temperature and usually takes at least ten minutes. If the room temperature is hot, you are advised to move the computer to a cooler location.
Step 1: Download CoreTemp from the official website.
Step 2: Launch the application and all the information of your processors is involved. Pay attention to the temperature readings .
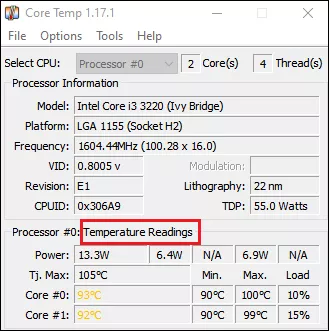
Keeping the fans running properly is the most essential step in getting your computer to cool down. Power off the computer and open your PC case, then you will first need to check that the fan blades are not damaged or not hung up on anything else. If much dust has gathered around the fan or vents, sweep it away since dust is sometimes the main cause of overheating. If the internal chassis looks both undamaged and clean, reconnect the power cable, but do not close the lid of the chassis so that good air flow can help it cool down. Then you need to turn on the computer to check if the computer fan, CPU fan and graphics card fan are spinning properly.
There is also a less common case where the processor overheats due to insufficient thermal paste between the CPU and its heat sink. Removing the old thermal paste and reinstalling the heat sink will be the only solution. Find a professional repair service if you are unable to perform this operation.
Step 1: Press Ctrl + Shift + Esc to open Task Manager.
Step 2: Choose Processes tab and find out the running processes of high CPU usage.
Step 3: Right-click on a program and select End task .
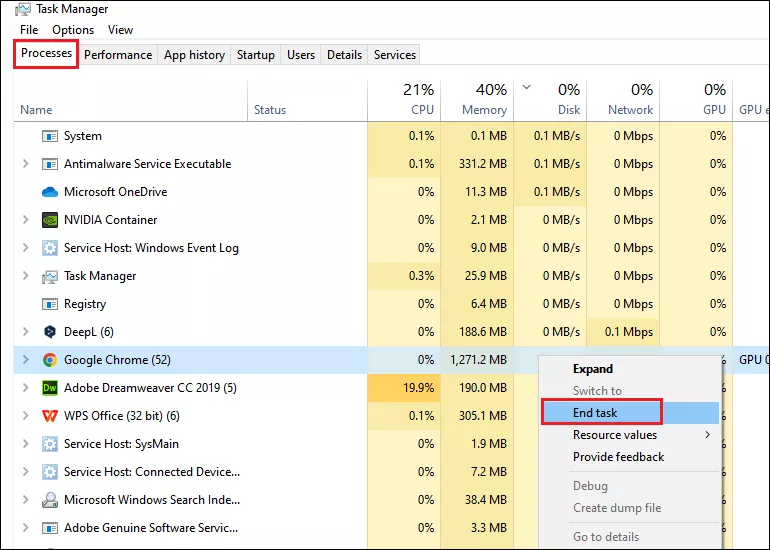
If your CPU is overheating because you have overclocked it, please stop doing so.
Step 1: Press Windows+I to open Settings and select Update & Security > Recovery . Then click on Restart now under Advanced startup.
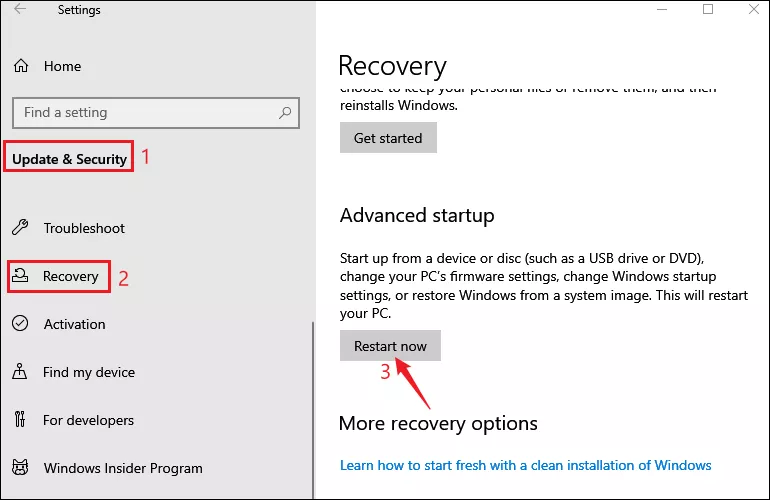
Step 2: Once it restarts, choose Troubleshoot option and select Advanced options .
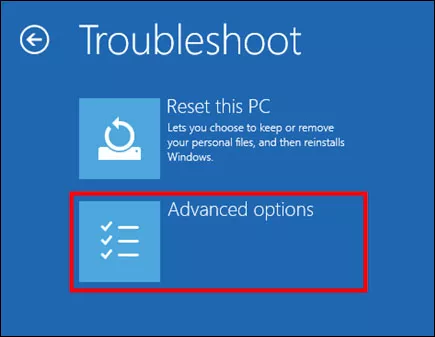
Step 3: Select UEFI Firmware Settings and click Restart .
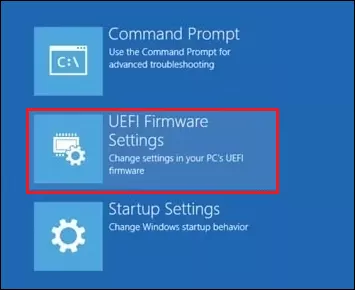
Step 4: Then the BIOS or UEFI menu will open, choose the Advanced tab and select Performance .
Step 5: Find an option marked as overclock or something similar and disable it.
Step 6: Press F10 to save the change and then restart your system.
When you check the CPU usage of the background programs in the fourth method, you find no programs are straining the system despite the high CPU usage, then it is likely that the memory is insufficient. Also read: RAM usage high: 12 ways to free up your RAM
Related Articles
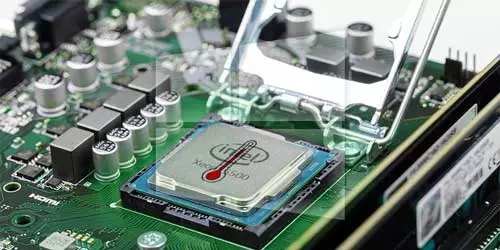
How to Check the CPU Temperature of Your PC in Windows 10
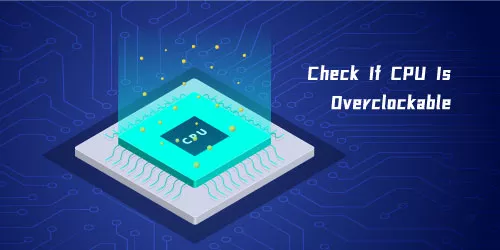
How to Check If CPU Is Overclockable: 4 Things You Need To Know
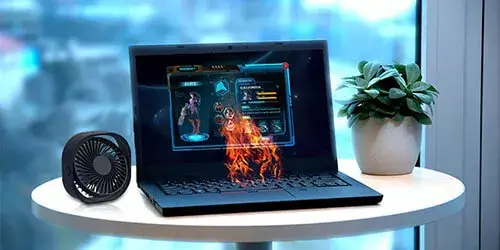
How to Cool Down My Laptop While Playing Games?
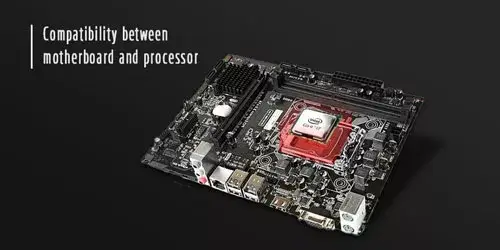
How to Know If A Processor is Compatible with A Motherboard (100% Workable)
Recent posts.

How to Check Who Is Accessing Shared Folder on Windows
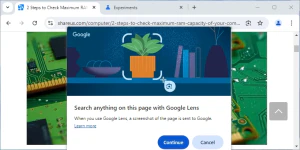
How to Enable and Use Google Lens in Chrome
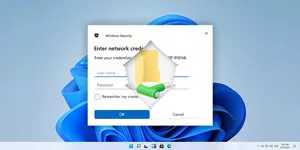
How to Share Folder Without Password on Windows 10 & 11
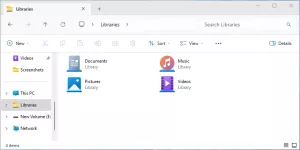
How to Efficiently Manage Your Libraries Folder
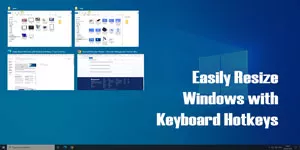
Easily Resize Windows with Keyboard Hotkeys
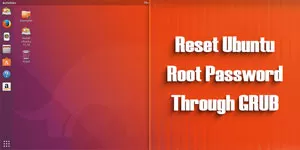
Reset Ubuntu Root Password Through GRUB
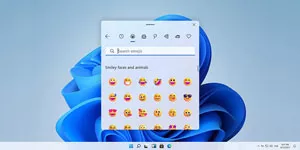

3 Ways to Type Emoji in Windows
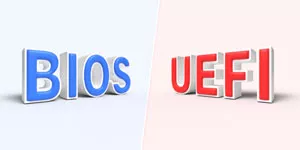
Understanding the Difference Between BIOS and UEFI
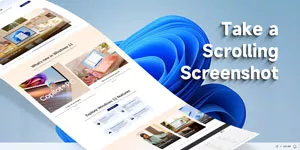
How to Take a Scrolling Screenshot in Windows
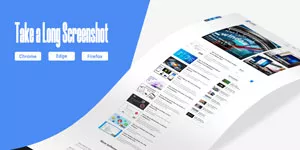
How to Take a Long Screenshot on Chrome, Edge, and Firefox?
Popular articles.
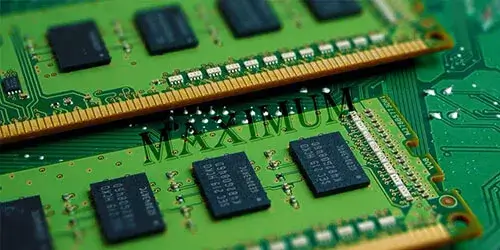
Two Steps to Check Maximum RAM Capacity of Your Computer
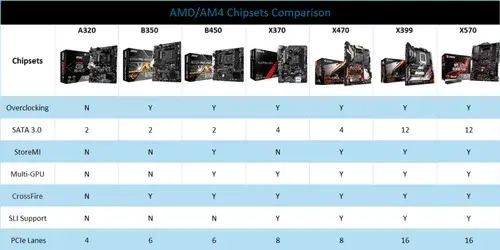
AMD/AM4 Chipsets Comparison: A320, B350, B450, X370, X470, X399 and X570
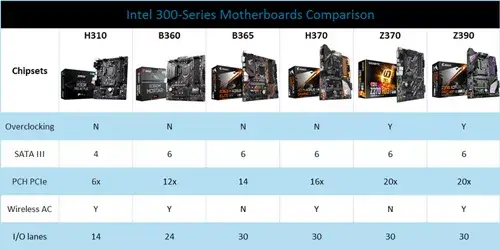
Intel 300-Series Motherboards Comparison: Intel H310, B360, B365, H370, Z370, Z390
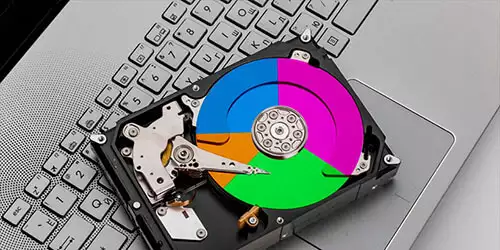
Recommended - Best Partition Size of 500GB Hard Disk for Windows 7, 8 and 10
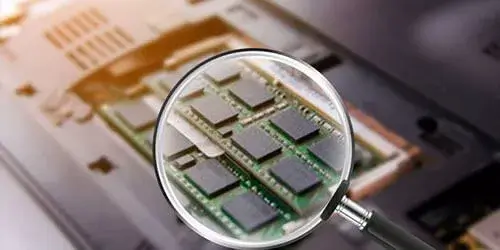
How to Check RAM Slots Number in Laptop - Windows 10/8/7
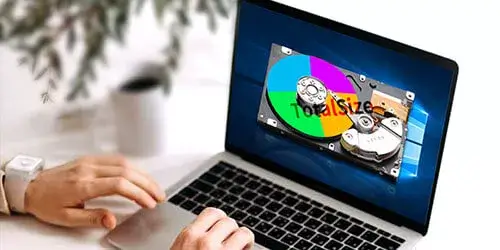
How to Check TOTAL Size of Hard Disk Space in Windows 10/8/7
Success! Subscription added.
Success! Subscription removed.
Sorry, you must verify to complete this action. Please click the verification link in your email. You may re-send via your profile .
- Intel Community
- Product Support Forums
Processor Thermal Trip
- Subscribe to RSS Feed
- Mark Topic as New
- Mark Topic as Read
- Float this Topic for Current User
- Printer Friendly Page
- Mark as New
- Report Inappropriate Content
- Intel® Q87Q85 Chipset
View solution in original post
- All forum topics
- Previous topic
Link Copied

Community support is provided Monday to Friday. Other contact methods are available here .
Intel does not verify all solutions, including but not limited to any file transfers that may appear in this community. Accordingly, Intel disclaims all express and implied warranties, including without limitation, the implied warranties of merchantability, fitness for a particular purpose, and non-infringement, as well as any warranty arising from course of performance, course of dealing, or usage in trade.
For more complete information about compiler optimizations, see our Optimization Notice .
- ©Intel Corporation
- Terms of Use
- *Trademarks
- Supply Chain Transparency
Help: Supermicro x11dpg-qt powers on and shuts down immediately
- Thread starter Bert
- Start date Mar 28, 2024
- DIY Server and Workstation Builds
Well-Known Member
I just got this motherboard. It comes with 2x Silver 4109T CPUs. Physically looks perfect. IPMI works but cannot login. Seller does not know the IPMI password and I am not sure where the default IPMI password is located. I am about to thrash it but I wanted to check here if there are any ideas on how to salvage it. Symptoms indicate dead motherboard: - CPU is not powering on. It stays cool. - MB starts and shuts down immediately or starts and hangs there. That keeps on changing. MB is responsive to the power button. - No display no beeps. - Network chip powers on. - SB felt cold but that may be normal. - Tried all the usual combos: Reseat CPUs, remove one CPU, change DIMMs. Didn't try to clear CMOS etc, not sure if that would help. It is sad to see it getting being thrashed. Any ideas on what to check and try?
I would probably try resetting CMOS, then the low-level BIOS flash from a USB stick, described on page 183 of the manual: https://www.supermicro.com/manuals/motherboard/C620/MNL-1998.pdf But I wouldn't be super optimistic.
nexox said: I would probably try resetting CMOS, then the low-level BIOS flash from a USB stick, described on page 183 of the manual: https://www.supermicro.com/manuals/motherboard/C620/MNL-1998.pdf But I wouldn't be super optimistic. Click to expand...
Yeah it requires some level of function but I have heard it fix some awfully broken sounding systems.
Is this a dead board or should I try a few other things like changing the CPU? I cannot flash the BIOS because it asks for activation code.
Looks like it is mistakenly reading the CPU temperature too high and refusing to start, I assume that sensor is on the CPU itself, so trying another might be worth it. You can pretty easily find instructions to generate a IPMI key if you want to go that way.
Active Member
Indeed, I'd try to remove the CPU that causes the Thermal Trip. This suggests that the system does in fact power on, but then immediately shuts down again as a safety measure. CPU1 might or might not be the one you've already removed during your tests. I assume you made sure to remove the correct CPU during your tests, which is the 2nd one. If they're numbered CPU0 and CPU1, then you've already removed the possibly faulty one. In this case it would be worth to do it again and check if there are other log entries when trying to power the system on like that. If they're numbered CPU1 and CPU2, then remove CPU1 and put CPU2 into the first socket and try powering it on.
I have extra CPUs that I know they work. On the other hand, it is possible motherboard is tripping because it has bad comms with CPU. I will circle back in a few weeks.
- This site uses cookies to help personalise content, tailor your experience and to keep you logged in if you register. By continuing to use this site, you are consenting to our use of cookies. Accept Learn more…
No results were found for your search query.
To return expected results, you can:
- Reduce the number of search terms. Each term you use focuses the search further.
- Check your spelling. A single misspelled or incorrectly typed term can change your result.
- Try substituting synonyms for your original terms. For example, instead of searching for "java classes", try "java training"
- Did you search for an IBM acquired or sold product ? If so, follow the appropriate link below to find the content you need.
Search results are not available at this time. Please try again later or use one of the other support options on this page.
CPU thermal trip errors in the BMC system event log - IBM System x3450
Troubleshooting.
The Baseboard Management Controller (BMC) System Event Log (SEL) contains repeated entries for Central Processing Unit (CPU) Thermal Trip events.
Resolving The Problem
RETAIN tip: H196750
Affected configurations
The system may be any of the following IBM servers:
- System x3450, type 7948, any model
This tip is not software specific. This tip is not option specific.
Additional information
There may be several reasons for this issue:
- There is not enough thermal grease between the processor and the heatsinks, or the heatsinks are not completely screwed down on to the system board.
- The processors were changed at some point from the time the replacement part numberSDR utility was run. It is recommended to re-run the replacement part numberSDR10 utility to reload the SDRs for these processors.
- Make sure that the air duct is properly installed prior to powering on the system.
- Make sure that the chassis cover is fully installed prior to powering on the system.
- When a CPU thermal trip error occurs, it is logged to the SEL and recorded in a CPU history log that Basic Input/Output System (BIOS) maintains. As long as the event remains in the CPU history log, the error will persist with subsequent reboots. Even if the user swaps out the processors with new ones, as long as the original event is still present in the history log, the error will persist and the CPU will remain offline. The only way to clear the error is to clear the history log. This is done by setting the "Processor Retest" option in the F2 BIOS Setup utility.
Document Location
Operating system.
System x:Operating system independent / None
Was this topic helpful?
Not useful Useful
Document Information
Modified date: 30 January 2019
ibm1MIGR-5083626
Page Feedback
The paradox of thermal vs. non-thermal effects in plasmonic photocatalysis
- Rishi Verma ORCID: orcid.org/0000-0003-0960-4460 1 ,
- Gunjan Sharma ORCID: orcid.org/0000-0002-4683-8488 1 &
- Vivek Polshettiwar ORCID: orcid.org/0000-0003-1375-9668 1
Nature Communications volume 15 , Article number: 7974 ( 2024 ) Cite this article
Metrics details
- Materials for energy and catalysis
- Photocatalysis
The debate surrounding the roles of thermal and non-thermal pathways in plasmonic catalysis has captured the attention of researchers and sparked vibrant discussions within the scientific community. In this review, we embark on a thorough exploration of this intriguing discourse, starting from fundamental principles and culminating in a detailed understanding of the divergent viewpoints. We probe into the core of the debate by elucidating the behavior of excited charge carriers in illuminated plasmonic nanostructures, which serves as the foundation for the two opposing schools of thought. We present the key arguments and evidence put forth by proponents of both the non-thermal and thermal pathways, providing a perspective on their respective positions. Beyond the theoretical divide, we discussed the evolving methodologies used to unravel these mechanisms. We discuss the use of Arrhenius equations and their variations, shedding light on the ensuing debates about their applicability. Our review emphasizes the significance of localized surface plasmon resonance (LSPR), investigating its role in collective charge oscillations and the decay dynamics that influence catalytic processes. We also talked about the nuances of activation energy, exploring its relationship with the nonlinearity of temperature and light intensity dependence on reaction rates. Additionally, we address the intricacies of catalyst surface temperature measurements and their implications in understanding light-triggered reaction dynamics. The review further discusses wavelength-dependent reaction rates, kinetic isotope effects, and competitive electron transfer reactions, offering an all-inclusive view of the field. This review not only maps the current landscape of plasmonic photocatalysis but also facilitates future explorations and innovations to unlock the full potential of plasmon-mediated catalysis, where synergistic approaches could lead to different vistas in chemical transformations.
Introduction
The phenomenon of localized surface plasmon resonance (LSPR) is a distinct occurrence arising from the collective oscillations of conduction-band electrons within metals like gold, silver, copper, and heavily doped semiconductor nanostructures 1 , 2 , 3 , 4 , 5 , 6 , 7 , 8 , 9 , 10 . These materials possess a distinctive capability to effectively interact with light, profoundly influencing their optical characteristics. By confining electromagnetic radiation to dimensions smaller than the wavelength of the incident light, LSPR enables the amplification of the local electromagnetic field. This, in turn, holds promising potential for advancing solar energy harvesting and photocatalysis 1 , 2 , 3 , 4 , 5 , 6 , 7 , 8 , 9 , 10 . When plasmonic nanoparticles are illuminated by light, an intriguing outcome is the creation of high-energy non-equilibrium electron-hole pairs. These energetic charge carriers referred to as “hot carriers,” exhibit properties that significantly deviate from the typical thermally equilibrated Fermi-Dirac energy distribution of the metal’s free electrons in thermal equilibrium. This departure from thermal behavior is what earned them the name “hot carriers” 1 , 2 , 3 , 4 , 5 , 6 , 7 , 8 , 9 , 10 .
The investigation into hot carriers has garnered significant attention across diverse disciplines, especially within the realms of nanotechnology and optoelectronics 11 , 12 , 13 , 14 , 15 , 16 , 17 , 18 , 19 , 20 , 21 , 22 , 23 , 24 , 25 , 26 , 27 , 28 , 29 , 30 , 31 , 32 , 33 , 34 , 35 , 36 , 37 , 38 , 39 , 40 , 41 , 42 , 43 , 44 , 45 , 46 , 47 , 48 , 49 , 50 , 51 , 52 , 53 . Their high-energy characteristics have the potential to bring about a paradigm shift in the way light interacts with matter, paving the way for groundbreaking applications like sensitive photodetection, efficient energy harvesting, and advanced photocatalysis 1 , 2 , 3 , 4 , 5 , 6 , 7 , 8 , 9 , 10 , 11 , 12 , 13 , 14 , 15 , 16 , 17 , 18 , 19 , 20 , 21 , 22 , 23 , 24 , 25 , 26 , 27 , 28 , 29 , 30 , 31 , 32 , 33 , 34 , 35 , 36 , 37 , 38 , 39 , 40 , 41 , 42 , 43 , 44 , 45 , 46 , 47 , 48 , 49 , 50 , 51 , 52 , 53 . Moreover, plasmonic nanoparticles possess a captivating ability to transfer these energetic hot carriers to nearby molecular adsorbates on their surface, triggering a range of electronic and chemical processes. This phenomenon in catalysis is commonly referred to as the “non-thermal” pathway of activation of molecules. Furthermore, as hot electrons participate in interactions with phonons (quantized vibrations in a material), they gradually dissipate their excess energy, causing the heating of the nanoparticle itself. This subsequent heat can then disperse into the surrounding environment, resulting in what is termed the photothermal effect or the “thermal” pathway of activation of molecules 1 , 2 , 3 , 4 , 5 , 6 , 7 , 8 , 9 , 10 , 11 , 12 , 13 , 14 , 15 , 16 , 17 , 18 , 19 , 20 , 21 , 22 , 23 , 24 , 25 , 26 , 27 , 28 , 29 , 30 , 31 , 32 , 33 , 34 , 35 , 36 , 37 , 38 , 39 , 40 , 41 , 42 , 43 , 44 , 45 , 46 , 47 , 48 , 49 , 50 , 51 , 52 , 53 .
Plasmonic nanoparticles have showcased their ability to enable a wide array of chemical transformations on their surfaces upon exposure to light for a range of reactions 1 , 2 , 3 , 4 , 5 , 6 , 7 , 8 , 9 , 10 . Yet, understanding the exact mechanism underpinning plasmon-induced chemical changes has sparked substantial deliberation. Within this discourse, two prevailing perspectives have come forward. One postulates that the transfer of hot carriers to adsorbed molecules stands as the key step, aligning with the “non-thermal” pathway 54 , 55 , 56 , 57 , 58 , 59 , 60 , 61 , 62 , 63 , 64 , 65 , 66 , 67 , 68 , 69 , 70 , 71 , 72 , 73 , 74 , 75 , 76 , 77 , 78 , 79 , 80 , 81 , 82 , 83 , 84 , 85 , 86 , 87 , 88 , 89 , 90 , 91 , 92 , 93 , 94 , 95 , 96 , 97 , 98 , 99 , 100 , 101 , 102 , 103 , 104 , 105 , 106 , 107 , 108 , 109 , 110 , 111 , 112 , 113 , 114 , 115 , 116 , 117 , 118 , 119 , 120 , 121 , 122 , 123 , 124 , 125 , 126 , 127 , 128 , 129 , 130 , 131 , 132 , 133 , 134 , 135 , 136 , 137 , 138 , 139 , 140 , 141 , 142 , 143 , 144 , 145 , 146 , 147 , 148 , 149 , 150 , 151 , 152 , 153 , 154 , 155 , 156 , 157 , 158 , 159 , 160 , 161 , 162 , 163 , 164 , 165 , 166 , 167 , 168 , 169 , 170 , 171 , 172 , 173 , 174 , 175 , 176 , 177 , 178 , 179 , 180 , 181 , 182 , 183 , 184 , 185 , 186 , 187 , 188 , 189 , 190 , 191 , 192 , 193 , 194 , 195 , 196 , 197 , 198 , 199 , 200 , 201 , 202 , 203 , 204 , 205 , 206 , 207 , 208 , 209 , 210 , 211 , 212 , 213 , 214 , 215 , 216 , 217 , 218 , 219 , 220 , 221 , 222 , 223 , 224 , 225 , 226 , 227 , 228 . In contrast, the second viewpoint argues that the nanoparticle’s local temperature assumes a pivotal role, aligning with the “thermal” pathway 229 , 230 .
Proponents of the “non-thermal” pathway contend that when plasmonic nanoparticles absorb light, they generate hot carriers whose energy transfers to nearby molecules, and initiate the chemical reactions. This energy transfer can overcome energy barriers necessary for specific chemical processes, thus facilitating catalysis. This viewpoint underscores the significance of non-equilibrium hot carriers as the primary driver behind plasmon-enhanced chemical reactions, with the nanoparticle’s temperature increase playing a subordinate role 54 , 55 , 56 , 57 , 58 , 59 , 60 , 61 , 62 , 63 , 64 , 65 , 66 , 67 , 68 , 69 , 70 , 71 , 72 , 73 , 74 , 75 , 76 , 77 , 78 , 79 , 80 , 81 , 82 , 83 , 84 , 85 , 86 , 87 , 88 , 89 , 90 , 91 , 92 , 93 , 94 , 95 , 96 , 97 , 98 , 99 , 100 , 101 , 102 , 103 , 104 , 105 , 106 , 107 , 108 , 109 , 110 , 111 , 112 , 113 , 114 , 115 , 116 , 117 , 118 , 119 , 120 , 121 , 122 , 123 , 124 , 125 , 126 , 127 , 128 , 129 , 130 , 131 , 132 , 133 , 134 , 135 , 136 , 137 , 138 , 139 , 140 , 141 , 142 , 143 , 144 , 145 , 146 , 147 , 148 , 149 , 150 , 151 , 152 , 153 , 154 , 155 , 156 , 157 , 158 , 159 , 160 , 161 , 162 , 163 , 164 , 165 , 166 , 167 , 168 , 169 , 170 , 171 , 172 , 173 , 174 , 175 , 176 , 177 , 178 , 179 , 180 , 181 , 182 , 183 , 184 , 185 , 186 , 187 , 188 , 189 , 190 , 191 , 192 , 193 , 194 , 195 , 196 , 197 , 198 , 199 , 200 , 201 , 202 , 203 , 204 , 205 , 206 , 207 , 208 , 209 , 210 , 211 , 212 , 213 , 214 , 215 , 216 , 217 , 218 , 219 , 220 , 221 , 222 , 223 , 224 , 225 , 226 , 227 , 228 . Conversely, supporters of the “thermal” pathway propose that the predominant influence on plasmon-assisted catalysis is the local temperature elevation of the nanoparticle. According to this viewpoint, absorbed light primarily heats the nanoparticle, substantially increasing its temperature. This increased temperature, in turn, promotes chemical reactions on the nanoparticle’s surface, leading to the observed enhancement in catalytic activity. While the non-thermal hot carriers might still contribute, they consider their role in the overall catalytic process secondary to the heating effect 229 , 230 , 231 , 232 , 233 , 234 , 235 , 236 , 237 , 238 , 239 , 240 , 241 , 242 , 243 , 244 , 245 , 246 , 247 , 248 , 249 , 250 , 251 , 252 , 253 , 254 , 255 , 256 , 257 , 258 , 259 , 260 , 261 , 262 , 263 , 264 , 265 , 266 , 267 , 268 , 269 , 270 , 271 , 272 , 273 , 274 , 275 .
The ongoing debate between the “non-thermal” and “thermal” pathways has spurred a flurry of research and investigations. Both sides have presented compelling evidence to substantiate their claims, resulting in an exploration of this field that is both interesting and challenging. As researchers went deeper into the fundamental mechanisms governing plasmon-assisted chemical processes, innovative experimental techniques, and theoretical models have emerged to provide insight into the intricate interplay between hot carriers and thermal effects. This review offers a comprehensive examination of LSPR fundamentals and their subsequent consequences on decay. We discussed about the instances of reactions influenced by non-thermal and/or thermal pathways. Additionally, we explore the experimental strategies employed by researchers to illuminate the intricate interplay between these pathways.
Localized surface plasmon resonance excitation and decay
In the realm of photophysics, chemists often initiate their investigations with a simplified grasp of distinct electronic and vibrational movements. The dynamics of excited states are elucidated by referencing radiative and nonradiative decay mechanisms. This foundational approach proves valuable for the photophysical attributes of semiconductor nanoparticles, encompassing phenomena like surface recombination of charge carriers. However, this viewpoint encounters limitations when dealing with metal nanostructures, primarily due to the high density of electronic states at the Fermi level.
To explore the interaction between light and matter within metals, researchers turn to Mie theory, a framework elucidating the optical response of a spherical particle 1 , 2 , 4 . Applying Mie’s theory necessitates the dielectric constants of both the particle and its surrounding environment as inputs. The dielectric constants of metals exhibit frequency-dependent variations and consist of real and imaginary components. Broadly speaking, the real component dictates the localization of the LSPR band, while the imaginary component governs the dephasing of this resonance 1 . In this section, we discuss the underlying fundamental theory governing the absorption of photons by metal nanoparticles and the subsequent processes governing energy relaxation 1 , 4 .
Collective charge oscillations
Plasmonic oscillation is sustained by conductive materials having a partially filled band, where quasi-free electrons react to external electric fields. As a result, the interband transitions, coinciding with the plasmonic resonance, also play a role in the dephasing process 4 .
When modeling the behavior of carriers within the conduction band, we begin by visualizing an infinite and boundless gas of free electrons. This conceptual framework seamlessly aligns with the characteristics of real-world metals as we transition to electron quasiparticles. These quasiparticles mirror free electrons but possess an effective mass (denoted as “ m ,” distinct from the vacuum mass “ m 0 ”) depending upon the curvature of the band. These electrons follow the Fermi-Dirac statistics, a statistical distribution that characterizes the energy distribution of particles at thermal equilibrium ( f F ) as:
Here T and k B are the temperature of the system and the Boltzmann constant, respectively. Within such a framework, the Fermi energy ( E F ) assumes a pivotal role. As temperatures increase beyond absolute zero, the electrons disperse across states possessing energies close to the Fermi energy, conforming to the distribution defined by Eq. ( 1 ). An equivalent picture can be described in terms of the linear momentum of electrons as it is directly related to the energy of electrons. The energy of free electrons is given by:
where p is the linear momentum and k is the electron wavenumber. When an external electric field from incident light is applied, it disrupts the equilibrium of the system, causing the electrons to acquire momentum along the axis aligned with the field’s polarization. This electronic motion, specifically the average velocity of electrons within the Fermi sea, is effectively explained by the Drude model. How this motion depends on the dielectric constant of the metal has a significant impact on the overall photophysics of the LSPR excitation 4 .
Dielectric constants of noble metals
The Drude model, often referred to as the free electron model, stands out as the simplest yet most realistic approach for determining the dielectric constant of a metal. In the Drude model, the conduction electrons are effectively treated as a gas of free particles 4 . The response of these electrons to an electric field, can be obtained by a solution to the equations of motion for a single electron and then scaling up by the number of electrons per unit volume. This approach leads to the resulting expression for the dielectric constant:
where ω p —plasma frequency and γ b —bulk damping constant. The damping constant can be related to the mean free path ( l ) of the electrons by γ b = v F / l where v F —Fermi velocity 4 . The plasma frequency is determined by the following equation:
where n —electron density, ε 0 —vacuum permittivity, and m e —effective mass of the electrons. In the case of small particles, surface collisions can lead to modifications in the damping constant:
Here, l eff represents the mean distance electrons traverse before encountering surface scattering, and A is a constant that depends upon the interaction between electrons and the surface. The Drude model effectively captures the behavior of dielectric constants within the near-infrared (near-IR) segment of the spectrum. However, as we venture into the visible and near-ultraviolet regions, the model encounters limitations attributable to the existence of interband transitions. These transitions introduce a frequency-dependent damping effect that cannot be adequately addressed within the confines of the original Drude model 4 . As a consequence, it becomes imperative to introduce an additional term in the dielectric constant to accurately account for the influence of these interband transitions:
where ε ib ( ω ) is the interband contribution.
Breaking down Eq. ( 7 ) into its real and imaginary components and taking into account that, at optical frequencies where ω ≫ γ , we obtain:
Equation ( 8 ) shows that for small particles ε 1 ( ω ) ≈ ε 1 bulk ( ω ), that is, the real component of the dielectric is the same as the bulk value as there is no size-dependent term. However, the imaginary component is modified by electron surface scattering and can be written as:
Consequently, the process of size reduction exerts a profound influence on the damping of electron motion, a consequence largely attributed to surface scattering 4 .
The decay of LSPR
The collective electronic oscillation sustained within a metallic particle during plasmonic resonance excitation results in the storage of energy within a relatively organized many-body state. As this coherent carrier motion interacts with either the material supporting it or its surrounding environment, the stored energy gradually dissipates, eventually transforming into heat. By incorporating plasmonic nanoparticles within a photocatalytic context, the goal is to establish a platform where other elements can efficiently harness this energy for subsequent chemical transformations. This will serve as a guide for the energy’s progression downstream in terms of its coherence.
The relaxation process can be dissected into distinct components unfolding across various timescales (Fig. 1a ). In the initial few femtoseconds (fs), the LSPR undergoes dephasing, triggering the generation of excited electron-hole pairs due to Landau damping and other interactions between photons and electrons. In this brief interval, the excited electron-hole pairs, characterized by a non-thermal distribution, decay either through photon re-emission (radiative) or via electron-electron interactions (non-radiative). Essentially, the energy initially in the form of photons gets transformed into electronic energy during this phase (Fig. 1a ). While the generation of hot carriers by this process at this stage is generally acknowledged, further experimental validation is essential. Moreover, in plasmonic NPs, hot carriers can be generated through both direct excitation (band transitions) and, particularly more effectively, via LSPR excitation and subsequent decay. The energy level of the resultant electron-hole pair is dictated by the incident photon’s energy, yet the energy dispersion is contingent upon the excitation mechanism. Plasmon decay yields higher-energy (hot) electrons and (cold) holes situated just below the Fermi energy, whereas direct excitation typically leads to cooler electrons and hotter holes. Subsequently, over a timespan ranging from 100 fs to several picoseconds, the excited carriers transmit their energy to electrons with lower energy levels through electron-electron interactions. This process leads to the eventual establishment of a quasi-Fermi-Dirac distribution for electron energy (Fig. 1a ). Eventually, the electron-hole pairs undergo relaxation, liberating thermal energy through electron-phonon interactions, a process occurring over a relatively protracted timescale extending up to hundreds of picoseconds to nanoseconds (Fig. 1a ). Consequently, the effects stemming from the excitation and relaxation of LSPR manifest as electromagnetic near-field enhancement, excitation of charge carriers, and localized heating effects 1 , 4 .
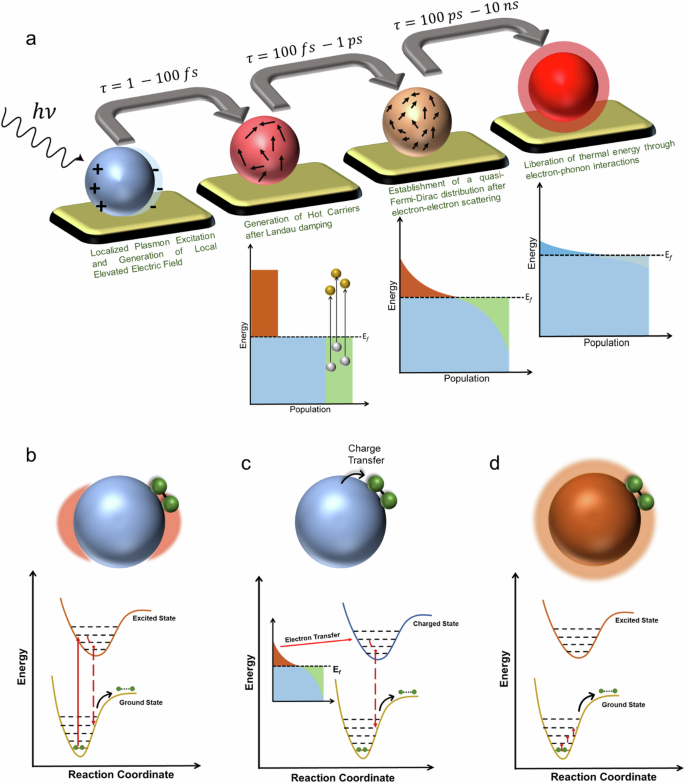
a LSPR excitation and the main effects induced by its relaxation. The time values represent the average time scales associated with each process and the energy diagram shows the changes in the band structure at each step. Plasmon-mediated chemical reactions, b electromagnetic near-field enhancement resulting in increase in the probability of molecular excitation near the plasmonic nanostructure, c transfer of excited charge carriers to the molecule near the surface, d local temperature increase and the increase in the population of molecules in vibrationally excited states.
Plasmon-mediated chemical reactions
Guided by the above discussion on fundamental processes of surface plasmon excitation and relaxation, in this section, we discuss a comparative analysis of plasmon-mediated catalytic reactions. It is crucial to emphasize that distinct types of LSPR effects often occur simultaneously, and the excitation and relaxation attributes of LSPR can be influenced by molecules adsorbed on the surface. Through comparison, we elucidate the distinct characteristics and physicochemical explanations that set the plasmon-mediated catalytic reactions apart from more comprehensively understood reactions. This may enable researchers to delineate the features and intricacies of plasmon-mediated catalytic reactions, contributing to a better comprehension of their mechanisms and outcomes (Fig. 1b–d ). The enhanced electromagnetic near-field fosters a significant augmentation in light absorption, resulting from intensified light intensity. This leads to an increased probability of exciting the reactant adsorbed on the plasmonic surface, a phenomenon routinely applied in surface-enhanced Raman spectroscopy (SERS). Such intensified excitation can even be experienced by the neighboring semiconducting interface in the case of a hybrid catalyst (Fig. 1b ). For instance, in the case of Au nanoparticles loaded onto the surface of an N -doped TiO 2 electrode, light irradiation demonstrated a 66-fold increase in the photocurrent for water splitting 54 . Conversely, ultraviolet-light illumination yielded a reduction in the photocurrent by a factor of 4 54 . In another analogous system involving Ag nanoparticles integrated into an N -doped TiO 2 photoanode, the enhanced photocurrent was attributed to the increased electromagnetic field at the interface between the Ag NPs and the N-doped TiO 2 . This inference was further substantiated by the dependence of the photocurrent on light intensity 55 . In both aforementioned instances, the LSPR exhibited by Au or Ag nanoparticles resulted in a heightened electric field proximal to the semiconductor’s surface. This augmented field amplified the probability of photon absorption within the semiconductor, consequently increasing the photocurrent. Nevertheless, a crucial factor for the successful completion of a reaction lies in the overlap between the absorption spectra of the plasmonic nanostructure and the reaction precursor or the semiconductor. Another possibility arises when plasmonic nanoparticles are photoexcited, reactant molecules attached to the NP surfaces are raised to higher electronic energy levels, facilitated by the charge transfer from the plasmonic NP to yield a transiently lived negative ion of the reactant molecule (Fig. 1c ). In this excited state, the reactant molecule undergoes reorganization, altering bond angles and lengths, which eventually triggers a chemical reaction. If the reactant doesn’t react during this initial excited state due to its short-lived nature, there’s a possibility for it to react in an excited vibrational state that possesses a relatively longer lifetime. Plasmonic nanostructures can also serve as localized heat sources, strategically influencing chemical reaction rates (Fig. 1d ). Traditional methods often struggle to confine thermal regions to the nanoscale, but plasmon-mediated heating offers a distinctive and promising solution. This characteristic sets plasmon-mediated heating apart, showcasing its potential for diverse applications in various reactions.
The preceding discussion on the possible pathways of chemical activation by plasmonic catalysis resembles a puzzle where each piece contributes to a coherent and sophisticated overall picture. Despite this, the precise mechanism of plasmon-assisted reactions remains ambiguous in much of the reported literature. Chemical reactions facilitated by plasmonic catalysis could theoretically follow any one or a combination of the discussed pathways, including near-field enhancement, charge transfer, or local temperature increase. The exact pathway chosen is contingent upon numerous factors, such as the nature of the metal NPs, the specific reaction and reactants involved, and surrounding parameters like dielectric properties, refractive index, band gaps, heat diffusion, and electron mobility 1 , 4 , 9 . The pathway followed by the process is significantly influenced by both the chemistry of the reactant molecules and the metal NPs, as well as the photophysics of LSPR. The probability of generating hot electrons hinges on the efficiency of electron-surface scattering, which is optimized in systems with surface boundaries that disrupt the translational symmetry of the material, facilitating electron scattering and photon absorption 1 , 4 . The subsequent creation of thermalized hot electrons through electron-electron interactions depends on the electron-electron scattering constant, while the conversion of light energy into heat relies on the electron-phonon scattering constant. These constants are greatly affected by the size of the metal NPs. For spherical particles, smaller NPs exhibit higher electron-surface scattering, leading to a greater probability of generating hot electrons and enhancing electron-assisted catalysis. Conversely, larger NPs exhibit stronger electron-phonon coupling, resulting in more pronounced photothermal effects 1 , 4 . Ideally, this information aids in designing and predicting the mechanisms of plasmonic reactions, but in reality, the process is more complex and less predictable. Despite the theoretical models and experimental insights providing a foundational understanding, practical applications often encounter challenges. The precise values of these coupling constants depend on various parameters, including size, shape, refractive index of the material and its surroundings, band structure, and the presence of interband and intraband absorption around LSPR frequencies. Although there is a clear and quantifiable correlation between different optoelectronic properties of the metal NPs and the relative contributions of thermal and non-thermal effects in catalysis, our understanding remains incomplete due to the lack of extensive experimental and theoretical studies. The complexity is escalated by the small size of NPs and the short lifetimes associated with LSPR. Additionally, the dependency of these properties on a wide range of parameters complicates the path to a definitive conclusion. However, some reports have successfully interwoven these properties to provide a clearer understanding of the intricate nature of plasmonic catalysis. We have discussed some of these results in detail in the later ultrafast section of this review.
Heat or “hot” electrons—why to bother?
Before discussing various examples from literature and potential mechanisms, it is essential to ponder: why does understanding the underlying mechanism of enhancement matter, when the input light energy effectively boosts the production rate of an industrially desired chemical through one or the other means? While the immediate response may suggest that comprehending the reaction mechanism aids in designing superior catalysts for specific reactions, the rationale runs deeper. To elucidate, let’s embark on a thought experiment focusing on a reaction happening in a solvent or medium. Consider a scenario where the activation mode of a reactant molecule by a plasmonic catalyst is solely thermal. Here, an immediate query arises: why utilize such a catalyst instead of conventional heating sources? There are two key advantages to employing plasmonic catalysts in this context. Primarily, it reduces reliance on non-renewable energy sources by harnessing abundant light energy, particularly sunlight, under the condition that the infrastructure is available. Secondly, photothermal heating of plasmonic materials offers highly localized heat, yielding a desirable spatial temperature profile unattainable with conventional methods. In such instances, the selection of the reaction medium is made based on its heat conduction and convection properties to sustain localized heating on the plasmonic particle.
Now, let’s pivot to a scenario where the activation of a reactant molecule by a plasmonic catalyst is purely non-thermal, facilitated by hot electrons. Here, considerations shift towards the redox properties of the medium. Optimal reduction potential and band positions become paramount to ensure minimal interaction between the medium and hot electrons. The dielectric constant of the medium plays a pivotal role, affecting the lifetime of charged intermediates potentially forming during the reaction. For an exothermic reaction catalyzed by hot electrons, a cooler reaction medium is favored to drive the reaction toward product formation. Conversely, for the same reaction catalyzed by purely thermal effects, cooling the reaction medium dissipates heat away from the plasmonic material, slowing reaction rates.
This examination highlights that maximizing catalyst potential necessitates understanding the underlying mechanism and shaping reactor design conditions accordingly. However, this pursuit is complicated by the intricate interplay between these effects, dictated by the photophysics of the material, catalyst type, whether the reaction occurs in liquid or gas phases, product selectivity, different reaction pathways in thermal and non-thermal conditions, electrode potential in case of electro-photo chemistry, light wavelength, and intensity. The varying thermal gradients, reactant diffusion times, dielectric properties, and other factors contribute further to this complexity. It is this complexity that instigates researchers’ curiosity to bother about whether it is the heat or hot electrons.
Plasmonic catalysis via non-thermal pathways
In this section, we highlight instances where researchers have found the non-thermal pathway as a key mechanism of plasmonic catalyst-mediated reactions. One noteworthy study, led by Linic et al., showcased the activation of oxygen molecules by Ag nanocubes when exposed to light. This activation prompted the partial oxidation of ethylene, yielding ethylene oxide 63 . The catalyst employed in this work was prepared by loading 20 wt.% of plasmonic Ag nanocubes (edge length of 75 nm) onto inert α-Al 2 O 3 particles (Fig. 2a ). The catalyst displayed high extinction of visible photons due to the presence of Ag nanostructures (Fig. 2b ). To unravel the intricacies of plasmonic activation, the reaction was conducted across varying temperatures and light intensities. Interestingly, the reaction rate exhibited a linear dependence with intensity up to approximately 300 mW/cm². Beyond this point, a superlinear power-law relationship emerged (Fig. 2c ). The power-law exponent reached a value of approximately 3.5 at an intensity of around 920 mW/cm². This observed transition from a linear to a super-linear regime with increasing intensity was considered a distinctive feature of electron-driven chemical reactions on metal surfaces 63 .
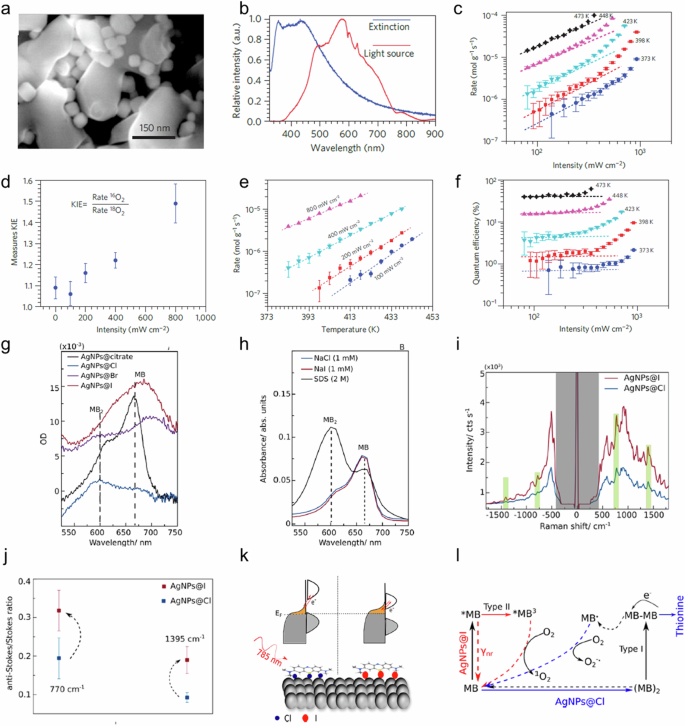
a Scanning electron micrograph of the catalyst containing densely packed Ag nanocubes supported on α-Al 2 O 3 , b diffuse reflectance ultraviolet-visible extinction spectrum of Ag nanocubes on α-Al 2 O 3 , c photocatalytic rate as a function of light intensity for various temperatures, d KIE, measured at constant reaction rate, as a function of source intensity, e photocatalytic rate (log scale) as a function of operating temperature for various source intensities, f quantum efficiency (%) as a function of intensity for various temperatures, g differential absorption spectra of MB adsorbed on as-synthesized AgNPs (capped by citrate), AgNPs@Cl, AgNPs@Br, and AgNPs@I at submonolayer concentration (500 nM, corresponding to approximately 0.5 MB molecules/nm 2 ), h optical absorption of free MB with Cl − , I − (the same concentration as in the SERS measurements, 1 μM) and sodium dodecyl sulfate (SDS) (/15) at an MB concentration of 50 μM, i anti-stokes/stokes SERS spectra of MB adsorbed on AgNPs@Cl and AgNPs@I, j anti-stokes/stokes ratio for the 1395 cm −1 vibrational modes of MB, k schematic representation of the energy levels of the AgNPs@Cl-MB and AgNPs@I-MB complexes, l pathways for methylene blue (MB) resonant photochemical reactions. a – f is reprinted with permission from ref. 63 , CCBY https://creativecommons.org/licenses/by/4.0/ , copyright 2012 Springer Nature without any changes. g – l is reprinted with permission from ref. 65 Copyright 2023 American Chemical Society.
The authors hypothesized a mechanism in which the excited electrons are formed after the LSPR decay and these excited electrons populate unoccupied adsorbate orbitals, creating the transient negative ion (TNI). The TNI moves around on its potential energy surface (PES). During this, the O–O bond stretches because the neutral O 2 molecule has a shorter equilibrium bond distance than the TNI. The relaxation of TNI happens by transferring back the electron to metal. This results in the O 2 being in its ground electronic but excited vibrational state. The authors assumed that the chemical reaction takes place when the vibrational energy is high enough to overcome the activation energy for the reaction. The hypothesis was also supported by the increase in the kinetic isotope effect (KIE), the ratio of the steady-state photocatalytic rate for the 16 O 2 and 18 O 2 isotopes, as a function of light intensity (Fig. 2d ). The enhanced KIE was the distinct signature of the non-thermal mode of activation of the reactions. The reaction rate also showed a positive relationship with light intensity and temperature, which was considered a signature of photocatalytic transformations on excited plasmonic nanostructures (Fig. 2e ). This was also reflected in the quantum efficiency of the reactions (Fig. 2f ). From these findings, the authors deduced that the transfer of excited electrons and the 3D arrangement of Ag NPs in the reactor bed played a crucial role in the shift from a linear to a super-linear rate dependence on intensity. This was particularly evident at relatively low light intensities and in the observed catalytic reactions 63 .
Cortés et al. also explored the dynamics of transferring hot electrons from plasmonic nanostructures to adsorbed molecules while also examining the influence of local electric field enhancement 65 . Their focus encompassed understanding how the conformation of adsorbed molecules and the metal-molecule state played pivotal roles in plasmonic chemistry. They elucidated the impact of co-adsorbed halide ions at the AgNPs-methylene blue (MB) interface on the selectivity of plasmon-driven N -demethylation of methylene blue to thionine. In addition, they probed the effects of light wavelength by utilizing both 632.8 nm and 785 nm (2 mW) wavelengths, resonating with exciting MB molecules and resonantly exciting the direct charge transfer between metal and molecule, respectively. They observed the influence of halide ions on MB adsorption behavior. They quantified the optical absorption of adsorbed MB molecules on AgNPs@Cl, AgNPs@Br, and AgNPs@I (Fig. 2g, h ). Intriguingly, Cl − ions were found to foster the formation of MB dimers on the AgNP surface, characterized by a distinctive optical absorption peak around 600 nm. In contrast, I − ions promoted the adsorption of MB monomers, manifested through an optical absorption peak at approximately 660 nm. On AgNPs@Br, a coexistence of both dimeric and monomeric forms was evident. The broadening of the absorbance peak observed in the case of MB on AgNPs@I and AgNPs@Br was attributed to inhomogeneous broadening arising from varying molecule-surface interactions 65 .
To further elucidate their findings, they utilized anti-Stokes/Stokes SERS, revealing that the rate of vibrational pumping of MB nearly doubled on AgNPs@I compared to AgNPs@Cl (Fig. 2i, j ). This was explained by the upward shift of the Fermi level of AgNPs caused by adsorbed I − ions. This shift played a crucial role in increasing the rate of direct transfer of charges between the metal and molecules on AgNPs@I. As a result, the rate of vibrational pumping increased substantially, leading to an elevated anti-Stokes/Stokes ratio (Fig. 2k ).
The authors made a notable understanding regarding the pivotal role of coadsorbed halide ions in determining both the conformation of adsorbed MB molecules and the energy levels of the AgNPs-MB complex. This dual influence governs the plasmon-assisted N -demethylation of MB to thionine. Their investigation revealed that when Cl − ions are coadsorbed on AgNPs, they induce the adsorption of MB as dimers, thereby enabling the resonant excitation of MB (632.8 nm) to proceed and driving the N -demethylation reaction. However, when I − ions are coadsorbed, MB adsorbs as monomers, leading to an alternate photochemical pathway where N -demethylation does not occur, instead, MB is excited to its triplet state. Despite having sufficient energy provided by plasmonic near-fields to potentially drive the MB demethylation reaction, the molecular conformation of adsorbed MB on AgNPs@I prevents the reaction from progressing. Under a distinct excitation condition at 785 nm, the energy of AgNPs’ Fermi level is elevated by I − ions. This enhancement favors the direct transfer of charges from the AgNPs to the adsorbed MB by chemical interface damping (CID). This results in an increased rate of vibrational pumping of MB molecules, yielding an enhanced anti-Stokes/Stokes SERS ratio. These findings also highlighted the critical significance of controlling energy and charge flow from plasmonic nanostructures to adsorbed molecules that depend on the nature of the hybrid state that is formed between the plasmonic metal and the molecule adsorbed. The conformation of the molecule adsorbed also played a critical role in the rate of reaction. This result highlighted the role of non-thermal effects in the catalysis because these effects would not play a major role in the reactions driven by purely thermal effects (Fig. 2l ) 65 .
Tian et al. in their work disentangled the effects of increased temperature from energetic charge carriers in plasmonic catalysis 62 . To achieve this, they constructed a plasmonic electrode integrated with photoelectrochemistry, allowing them to quantitatively discern between thermal and non-thermal effects. The plasmonic electrode was comprised of a uniform, large-area bowtie array of Au nanoelectrodes (Fig. 3a ), with each nanoelectrode exhibiting a diameter of 250 nm and a height of 100 nm, as determined by Atomic Force Microscopy (AFM) (Fig. 3b ). This electrode setup permitted the application of both negative and positive potentials, thus facilitating oxidation and reduction reactions under incident light. The plasmonic electrode displayed characteristic plasmon resonances around 560 nm and 650 nm, attributed to the LSPR of the bowtie and the surface plasmon polariton (SPP) of the periodic array, respectively (Fig. 3c ).
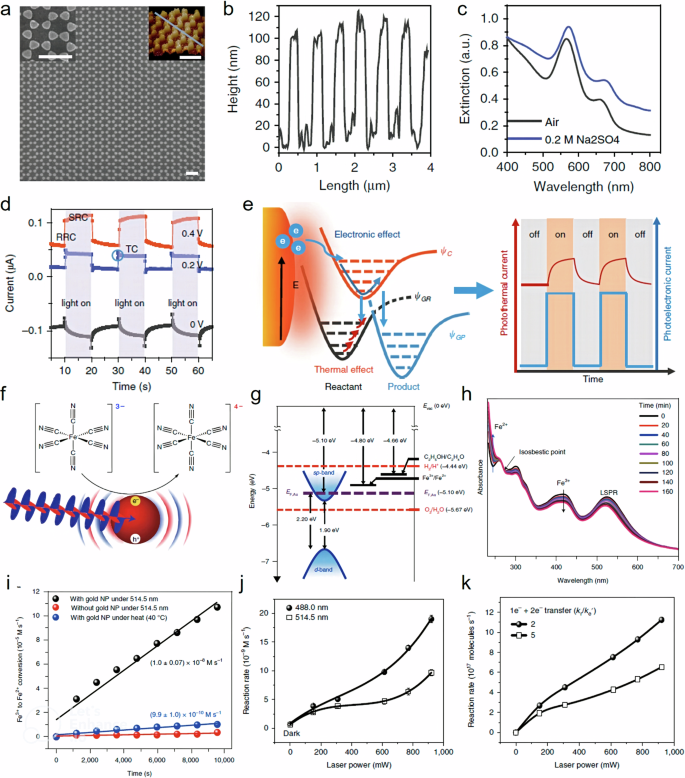
a SEM and AFM images, b the height profile of the as-prepared Au nanoelectrode array along the gray line in AFM image of ( a ), c the UV–Vis distinction spectrum, d the current-time curves of the Au nanoelectrode array, e the mechanism for the photoelectronic effect and photothermal effect of SPs on the electrochemical reaction, f The experimental model system comprising a gold NP photocatalyst and a Fe 3+ electron acceptor, g schematic of energy levels with absolute electrochemical energies, E , shown relative to the vacuum level: E (eV) = −4.44 eV − E SHE (eV), h UV–Vis spectra as a function of irradiation time show the quantitative conversion of Fe 3+ to Fe 2+ , i Fe 3+ to Fe 2+ conversion as a function of irradiation time (black dots), j plot of the photocatalytic reaction rate as a function of the incident laser power for two excitation wavelengths: 514.5 nm and 488.0 nm, k for the semi-quantitative model with 1e − and 2e − transfer reactions included, the calculated reaction rate as a function of laser power is shown for two different e - -h + recombination rates ( \(k_{{\rm{r}}}/k_{{\rm{e}}}^{\prime}=2 \;{{\rm{and}}}\; 5\) ). a – e is reprinted with permission from ref. 62 , CCBY https://creativecommons.org/licenses/by/4.0/ , copyright 2019 Springer Nature without any changes. f – k is reprinted with permission from ref. 80 , CCBY https://creativecommons.org/licenses/by/4.0/ , copyright 2018 Springer Nature without any changes.
In the photoelectrochemical study, the resulting photocurrent curve exhibited two distinct regions: a rapid response current (RRC) region operating on a timescale of approximately 0.05 s and a slow response current (SRC) region, which occurred over a timescale of about 10 s (Fig. 3d ). Moreover, a momentary electric current lasting less than 0.5 s appeared right after the RRC process, specifically when the SRC was restrained at an applied potential of 0.2 V. They tried to understand the reaction mechanism through a series of experiments involving temperature variations, applied potential, light intensity, wavelength, and the removal of oxygen from the solution by de-aeration. Their investigation yielded intriguing insights: the observed RRC was ascribed to excited carriers within the plasmonic electrode, while the SRC was linked to photothermal effects. The experimental photocurrent curve underscored the fact that both the photothermal and photoelectronic effects stemming from LSPR can influence chemical reactions and responses across distinct timescales. Furthermore, the photoelectrochemical approach allowed for a quantitative disentanglement of these effects (Fig. 3e ) 62 .
Jain et al. aimed to explore how efficiently and under what circumstances a plasmonic photocatalyst can capture multiple charge carriers in their study 80 . To achieve this, they studied the plasmon-induced electron transfer process in gold nanoparticle photocatalysts. This involved the one-electron (1e − ) reduction of ferricyanide ([Fe(CN) 6 ] 3– ) to ferrocyanide ([Fe(CN) 6 ] 4– ) (Fig. 3f, g ). Polyvinylpyrrolidone-capped Au NPs (13 nm), served as the photocatalyst. These nanoparticles exhibited plasmon absorption in the visible region and demonstrated stability against photooxidation (Fig. 3h ). Monochromatic visible light was utilized to activate the plasmon resonance of the gold nanoparticles. During visible-light exposure, the absorbance at 419 nm due to Fe 3+ decreased. Simultaneously, the absorbance at 240 nm, due to Fe 2+ , increased (Fig. 3h ). An isosbestic point at around 270 nm indicated the stoichiometric conversion of Fe 3+ to Fe 2+ . Monitoring the amount of Fe 3+ consumed (or the amount of Fe 2+ formed) over time directly reflected the number of electrons harvested from the gold nanoparticles (Fig. 3i , black dots) 80 .
The influence of hole scavengers was examined by examining various concentrations of these scavengers. It was found that the overall quantum efficiency of the reaction process was significantly influenced by the redox potential of the hole scavenger. When no hole scavenger was employed, the reaction rate reached saturation at a higher photon flux. However, when using ethanol as a hole scavenger, the reaction transitioned from a one-electron-hole pair transfer reaction to a multiple electron-hole pair transfer reaction at higher photon flux. This transition occurred due to the efficient utilization of holes by EtOH, which prevented the recombination of charge carriers. The researchers also varied the wavelength of light excitation, comparing plasmon-resonant excitation at 514 nm with interband excitation at 488 nm for gold nanoparticles. Upon modeling the kinetics of the reaction, they found that the rate was consistently higher for the 488 nm excitation across all laser powers. The difference in rates was most pronounced in the multiple electron transfer regime, where the rate for 488 nm excitation was twice that for 514 nm excitation. Additionally, the onset of the multiple electron transfer regime occurred at a lower power for the 488.0 nm excitation 80 .
The authors then attributed these observations to the fact that interband electron-hole pairs generated through d → sp transitions have longer lifetimes than those produced by intraband transition following plasmon-resonant excitation. This conclusion was supported by simulation results (Fig. 3j, k ), by plotting the (1e – + 2e – ) reaction rate against laser power for different electron-hole recombination rates in the model. By comparing the simulated plots with experimental trends, they confirmed that interband excitation aligned well with simulations using lower recombination rates, while plasmon-resonant excitation correlated with higher recombination rates. They concluded that the catalytic process hinged on generating a charge-separated state through interband transitions and effectively capturing holes using a scavenger. By employing suitable conditions—such as interband excitation, higher photon flux, and rapid hole scavenging—a multi-electron reaction could be achieved. Importantly, they highlighted that this multiple electron transfer regime could be attained using continuous-wave excitation rather than pulsed light sources highlighting another evidence of non-thermal effects in plasmonic catalysis 80 .
Halas et al. conducted a study aimed at examining the quantification of hot carriers and thermal influences in plasmonic photocatalysis 81 . They utilized a plasmonic antenna-reactor (AR) configuration composed of a Cu NP antenna coupled with Ru reactor sites on a photocatalyst surface alloy (Fig. 4a ). They focused on assessing the impact of optical illumination on the apparent activation energy barrier (Ea) in the process of ammonia decomposition. They explored various parameters, such as the wavelength and intensity of illumination, and concurrently monitored the surface temperature (Ts) of the photocatalyst pellet using thermal imaging to consider photothermal effects. The photocatalytic reaction rate on the Cu-Ru-AR surface alloy demonstrated an enhancement, approximately 20 times higher than individual Cu and 177 times higher than individual Ru nanoparticles (Fig. 4b ). Under an illumination intensity of 9.6 W cm −2 and in the absence of external heating, the rate of NH 3 decomposition over the Cu-Ru-AR configuration reached a H 2 production value of 1200 µmol g −1 s −1 . The catalyst remained active in five light on-off cycles (Fig. 4c ). Furthermore when the decomposition of NH 3 was carried out without illumination but under external heating conditions equivalent to those achieved during illumination, the thermocatalytic rates of H 2 production were lower, ranging from one to two orders of magnitude below the rates observed during photocatalysis (Fig. 4d ) 81 .
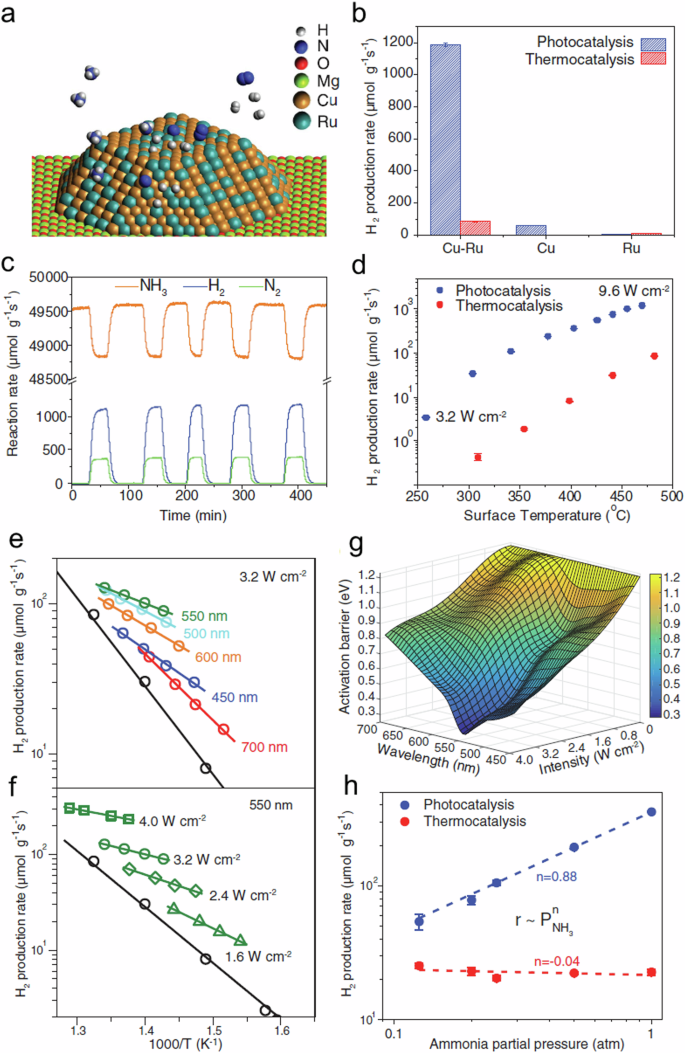
a Schematic of the structure of Cu-Ru-AR, b H 2 formation rate of photocatalysis (9.6 W cm −2 ) and thermocatalysis (482 °C), c multiple-hour measurement of photocatalytic rates, d comparison of photocatalytic and thermocatalytic rates on Cu-Ru-AR, Arrhenius plots of apparent activation barriers for e different wavelengths and f various light intensities at 550 nm, g a 3D representation of E a ( λ , I ) for different wavelengths and intensities, h reaction order with respect to P NH3 in photocatalysis (6.4 W cm −2 white light) and thermocatalysis (427 °C). The figure is from ref. 81 . Reprinted with permission from AAAS ( https://www.science.org/doi/10.1126/science.aat6967 ).
To assess the impact of illumination on the activation energy (Ea), they varied the chamber temperature for each combination of wavelength ( λ ) and intensity ( I ), thereby covering a spectrum of surface temperatures. By measuring the reaction rates and corresponding surface temperatures (Ts) under different conditions, they utilized the Arrhenius equation to deduce Ea( λ , I ). In Fig. 4 , panels e and f illustrate the outcomes: panel e displays the wavelength-dependent behavior of Ea at a constant intensity of 3.2 W cm −2 , while panel f portrays the intensity-dependent tendencies of Ea at a fixed excitation wavelength of 550 nm, aligning with the LSPR frequency of the Cu-Ru-AR photocatalyst.
When illumination was absent, Ea was measured at 1.21 eV. However, under resonance illumination at an intensity of 3.2 W cm −2 , the most significant reduction of Ea occurred, plummeting from 1.21 to 0.35 eV. As the wavelength increased, the decrease in Ea became less prominent due to diminished optical absorption, which consequently resulted in a decline in the generation of hot carriers. Conversely, at shorter wavelengths, the absorption remained considerable, attributed to Cu interband transitions, yet the reduction in Ea was comparatively minor. This discrepancy can be attributed to the distinct energies of energetic electrons produced by interband transitions in Cu, which are lower than those generated by plasmon decay. This contradicted previous findings suggesting that a larger number of less energetic carriers generated by interband transitions in Au nanoparticles were more efficient compared to those induced by plasmon resonant excitation in solution-phase catalysis 82 . However, it is important to acknowledge that the specific energetics intrinsic to the reaction itself likely play a pivotal role in determining photocatalytic efficiencies.
In the context of resonant illumination (Fig. 4f ), as light intensity increased, Ea displayed a decreasing trend. The three-dimensional contour map (Fig. 4g ) showcasing light-induced changes in Ea, revealed a consistent wavelength dependence across all light intensities, demonstrating that the pattern of decreasing Ea with rising intensity applied to all explored wavelengths. Under the optimal illumination conditions, occurring at the LSPR frequency and an intensity of 4 W cm −2 , Ea was successfully lowered to approximately 0.27 eV. Moreover, the elevation in the reaction order for NH 3 in the presence of light indicated the significant influence of hot carriers on the energetics of adsorbed nitrogen (N), finally promoting the net reaction rate by facilitating the N 2 desorption process (Fig. 4h ) 81 .
Frontiera et al. 97 used ultrafast nanoscale Raman thermometry to probe the effective temperature, equivalent to the mode-specific increase of vibrational kinetic energy, of molecules adsorbed on Au nanoparticles on the picosecond time scale (Fig. 5a ). They measured Stokes and anti-Stokes Raman spectra as a function of time, and from there they estimated the absolute temperatures for the non-equilibrium system using a Boltzmann distribution analysis. They used aggregates of Au NPs having the broad plasmon resonance response in the NIR region of the spectrum (Fig. 5b ). As test molecules, they used 4-nitrobenzenethiol (4-NBT) and trans-1,2-bis(4-pyridyl)ethylene as analytes which were adsorbed on the Au NPs. The Au NPs aggregates were excited with a femtosecond pump pulse, centered at 1035 nm, and a picosecond Raman probe pulse was used at various time delays to get a SERS spectrum. The intensity of the Stokes spectra was approximately 40 times larger than the anti-Stokes spectra for the 4-NBT molecule (Fig. 5c, d ). The obtained changes between the transient pump-on spectra were relatively small. These minor changes suggested that the effective temperature change between each time point is small relative to the vibrational frequency. They further analyzed the data and fitted the obtained intensities at various time points into a Gaussian function to extract the amplitude. This amplitude was then used to get an estimate of the local temperature. On comparing the baseline effective temperature and the corresponding transient temperature change of various modes relative to room temperature, it was found that the 1340 cm −1 mode (symmetric NO 2 ), had the strongest coupling with the plasmon, resulting in the highest baseline temperature, whereas the temperature increase in case of 1079 and 1578 cm −1 was small (Fig. 5e ). Another important observation from this study was that each mode of the adsorbate had similar time scale of coupling with plasmon and receiving the energy. In the case of trans-1,2-bis(4-pyridyl)ethylene, a similar increase in the transient effective temperature was observed in the two modes (Fig. 5f ). However, the magnitude of temperature increase was different for both the test molecules. This was attributed to the different coupling of each molecule with the plasmon. In this study, the temperature increase that was observed for both molecules was in the order of tens of Kelvin and this deposited energy depletes very rapidly, even after high photoexcitation values. This small increase in temperatures indicated that in the case of plasmon-driven reactions, thermal effects are minimal and a major role is played by the non-thermal mode of activation 97 . However, in another study, Baldi et al. also used nanoscale thermometry using β-phase copper phthalocyanine (β-CuPc) to measure the surface temperature of plasmonic gold nanoparticles under laser irradiation 100 . In this study they found an intensity-dependent temperature increase in the range of 300–450 K range with intensity varying from 0.5 to 4.5 W cm −2 .

a Scheme depicting ultrafast Raman thermometry of plasmonic photocatalysts. Using a femtosecond pulse, the authors excite the plasmon and then obtain Stokes and anti-Stokes surface-enhanced Raman spectra using a picosecond probe pulse at various time points during this process, b Extinction spectrum of the aggregated colloidal gold nanoparticle sample with adsorbed 4-nitrobenzenethiol (inset: SEM image). Ultrafast surface-enhanced c anti-Stokes and d Stokes Raman spectra of 4-nitrobenzenethiol adsorbed to colloidal gold nanoparticles. Comparison of effective baseline temperature (dark shading) and transient temperature change (lighter shading) relative to room temperature for e three modes of 4-nitrobenzenthiol, and f for two modes of trans-1,2-bis(4-pyridyl)ethylene. The figure is reprinted with permission from ref. 97 . Copyright 2018 American Chemical Society.
Kim et al. probed the effect of light excitation on the anti-Stokes Raman spectra of the 4-nitrobenzenethiol (NBT) molecules placed in between Ag nanoparticles and Au thin film 101 . They observed that the νNO was in the overtone-excited state as the anti-Stokes νNO peak of NBT showed a notable asymmetric broadening toward the lower frequency (Fig. 6a, b ), which was absent in the Stokes νNO 101 . On fitting several anti-Stokes spectra with Lorentzian profiles, the average population distribution indicated that 26% of the νNO-excited NBTs were in v νNO ≥ 3 with internal energy greater than 0.5 eV (Fig. 6c ). To understand the effect of light excitation, the authors employed electron−molecule scattering model because the photothermal heating cannot selectively excite a particular vibrational mode and a direct optical electronic excitation followed by rapid non-radiative decay are known to selectively excite the δNO but not the νNO mode. In the case of the electron–molecule scattering model, the electron is initially transferred to the neutral molecule, which results in the creation of a transient negative ion. This transient negative ion then re-emits the electron back to the metal and results in vibrationally excited states (Fig. 6d ). The energy required for the transfer of an electron to NBT to convert it into NBT •− was found to be around 0.4 eV as defined by the crossing point between the two potential energy surfaces of NBT and NBT •− (Fig. 6e ). The authors concluded that this energy was readily accessible by the energies of NBTs (v νNO ≥ 3). They also obtained a good correlation between the rate of the reaction and the degree of νNO-excitation (ρ νNO ), corroborating the possibility (Fig. 6f ). This study established the role of hot electron-mediated chemistry by using mode-selective multi-quantum vibrational transitions which were supported by the quantum theory of the hot electron-mediated molecular excitation 101 .
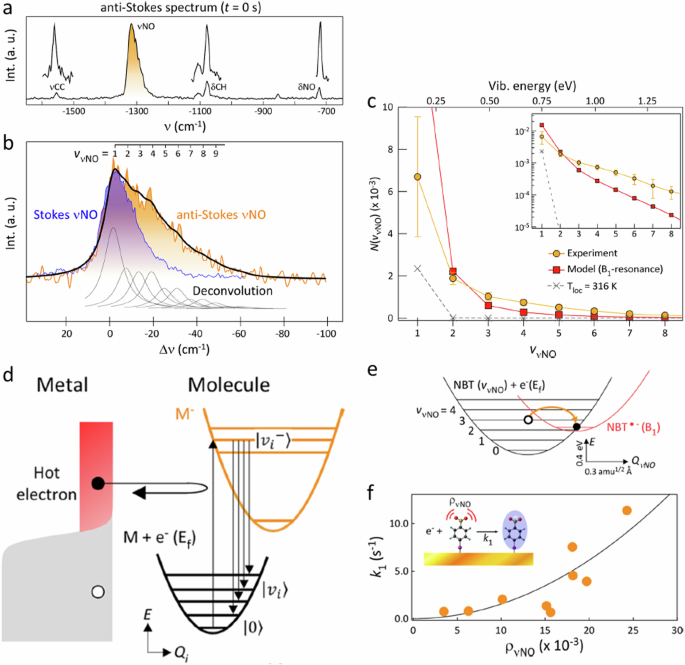
a An initial ( t = 0 s) anti-Stokes spectrum of NBT in a hotspot, showing the asymmetric broadening of the νNO peak (orange shading), b Anti-Stokes νNO peak of NBT (orange) obtained with a high-resolution spectrometer, and the deconvolution of the lineshape (a thick black curve) into multiple anti-Stokes peaks (thin solid curves in black) of vνNO → vνNO −1 transitions of NBT with vνNO = 1–11. Also shown as a comparison is the Stokes SERS νNO (blue) of NBT in a hotspot. The negative frequency (Δ ν < 0) corresponds to the red shift with respect to the expected center frequency of the mode, c N(v NO), the vνNO-population distribution of NBT (filled circles in orange) extracted from the lineshape analysis of the anti-Stokes SERS peaks of νNO. The inset shows the log-scale representation of the same data. The model (B 1 -resonance) distribution (squares in red) and the thermalized distribution at T loc = 316 K (crosses and dashed line in black) are also shown, d Electron–molecule scattering model, e Enlarged view of the harmonic potential energy curves for NBT + e − (black) and NBT •− (B 1 ) (red) along the νNO coordinate (redraw of curves). Approximate vibrational energy levels are also drawn in horizontal lines, f Correlation (filled circles) between the single-electron reduction rate (k1) for NBT + e − → NBT •− and the degree of νNO-excitation ( ρ νNO ) evaluated from SERS trajectories. The figure is reprinted with permission from ref. 101 . Copyright 2023 American Chemical Society.
Baldi et al. in another work investigated the role of thermal, and electron transfer by interband and intraband excitation in the light-driven Au@Ag core@shell nanorod (NR) synthesis 102 . Under the illumination of light of different wavelengths, they were able to establish the major role of interband excitation in Au that resulted in faster growth of the Ag shell. Three conditions were chosen for the growth of the Ag shell around the Au nanorods, dark, excitation by a 532 nm (240 mW) laser, and excitation by a 730 nm (55 mW) laser. They studied the growth kinetics by tracking the changes in the longitudinal plasmon resonance in all conditions. In the case of dark and 730 nm light excitation, no significant plasmon-driven Ag shell growth occurred, whereas the longitudinal peak blue shifted from 743 to 721 nm in 2 h, followed by a 14 nm red shift in the next 5 h, and finally, at the end of 24 h a blue shift of 65 nm occurred (Fig. 7a–e ). On estimating the temperature increase due to the photothermal heating, it was found that the temperature increase was <1 K as observed by the infrared camera and thermocouple measurements due to the high thermal gradient available in the solution phase due to high heat transport via conduction and convection. Hence, the photothermal effect did not play a major role in this reaction. Similarly, on calculating the electric field enhancement by FDTD simulation, the time evolution of enhancement was in sharp contrast with the experimentally measured decrease in rate enhancement throughout the reaction which allowed the ruling out of the near fields as the dominant contributor. The excited charge carriers that were formed after 730 nm light excitation didn’t have sufficient energy to carry out the reaction which was not the case in 532 nm light excitation. Further investigation of the reaction mechanism was carried out by measuring the angular distribution of these charge carriers which suggested that the d-band holes in gold were the main driving force behind the silver shell growth because the holes were scavenged through the oxidation of ascorbic acid (Fig. 7f–h ). This study is another example of a reaction in which the role of non-thermal effects was established 102 .

Time evolution of the extinction spectra of Au@Ag core@shell nanorods over a period of 24 h a in the dark, b under 55 mW, 730 nm laser illumination, and c under 240 mW, 532 nm laser illumination, d Time evolution of the longitudinal plasmon resonance under different illumination conditions, e Rate enhancement of silver shell growth under 240 mW, 532 nm (green) illumination compared to the experiment in the dark, plotted as a function of the reaction time under 532 nm illumination. Hot hole-assisted silver shell growth. Schematic representation of the energy levels for the plasmon-driven oxidation of ascorbic acid (AA) to dehydroascorbic acid (DHAA) and the concomitant reduction of Ag + to Ag 0 on gold nanorods under f dark conditions, g 730 nm illumination, and h 532 nm illumination. The dashed lines in ( g , h ) represent the average energy of nonthermalized holes under plasmon excitation. The dotted line in ( h ) represents the average energy of nonthermalized intraband holes, which are the only ones contributing to the plasmon-enhanced reactivity for shell thicknesses of >4 nm. The figure is reprinted with permission from ref. 102 . Copyright 2020 American Chemical Society.
Khatua et al. investigated the influence of simple organic ligands on the separation and utilization of plasmonic hot electrons generated via resonant excitation of triangular prism-shaped gold nanoparticles (AuTP) 138 . They functionalized the AuTP with various p-substituted benzene thiol (X-Ph-SH, where X = NO 2 , F, Cl, Br, H, OH, OCH 3 ) ligands and assessed their efficacy in driving the hydrogen evolution reaction (HER) from water at neutral pH under near-infrared (NIR) (808 nm) illumination. Their investigation revealed the significant role of surface dipole moment and orbital interactions between nanoparticles and ligands in HER rate. The AuTP-coated glass substrate exhibited a broad extinction spectrum covering visible and NIR wavelengths with an LSPR peak at 790 nm (Fig. 8a ), while SEM images depicted uniform deposition of AuTP (Fig. 8b ).

a UV–visible extinction spectra of AuTP attached to the glass substrate. The inset shows the photo of the AuTP functionalized glass substrate, b SEM image of the AuTP functionalized on ITO substrate. The scale bar is 100 nm, c The H 2 production rate and d the calculated incident photon to hydrogen conversion efficiency (IPHCE) for each substrate. The experiment was performed in neutral water under 808 nm laser illumination with a power density of 100 mW cm −2 , e Photocatalytic H 2 production was observed over time for ortho-, meta-, and para-substituted benzene-thiols and with 4-Br-Ph-CH 2 -SH functionalized AuTP substrates, f Comparison of the H 2 production rate observed with different Br substituted substrates, g Correlation between the dipole moment of the Au-ligand system for various substituents and hydrogen production rates, h Correlation between the combined function comprised of dipole moment and J with net hydrogen production rate by different Au-ligand systems. The figure is used with permission of S. Khatua, from ref. 138 ; permission conveyed through Copyright Clearance Center, Inc.
During photocatalytic HER testing, bare AuTP and AuTPs coated with F/Cl/NO 2 substituted benzene thiols exhibited minimal activity. In contrast, AuTPs with H/OH/OCH 3 substituted benzene thiols demonstrated a notable increase in HER rate, with AuTPs coated with Br-substituted benzene thiols showing the highest performance, producing hydrogen at a rate of 4.1 mL g −1 h −1 (Fig. 8c ). This rate was approximately seven times higher than AuTPs with H-Ph-SH ligand and approximately 4500 times higher than bare AuTPs (Fig. 8c ). The HER rates among AuTPs coated with different substituted benzene thiols followed the order: bare ≈ Cl ∼ F < NO 2 < H < OH < OCH 3 < Br, with a similar trend observed for the incident photon to hydrogen conversion efficiency (IPHCE) (Fig. 8d ). Confirmation of the role of hot electrons was achieved by adding a hole scavenger (10% methanol), resulting in a 1.4-fold increase in HER activity. To understand the effects of ligand substitution the authors replaced the 4-Br-Ph-SH ligand with 4-Br-Ph-CH 2 -SH and its ortho-, and meta-substituted counterparts. They found that the position of Br in the ligand played an important role in the HER activity. The HER rate decreased by approximately ∼ 3 folds when the 4-Br-Ph-SH ligand was replaced with 4-Br-Ph-CH 2 -SH (Fig. 8e, f ). Also, the rate decreased in the case of ortho- ( ∼ 90 folds), and meta-substituted ( ∼ 200 folds) bromobenzene thiols, and negligible hydrogen was obtained (Fig. 8e, f ). This underscored the crucial role of the Br atom’s position in HER activity.
They studied the correlation between the dipole moment of the Au-ligand system (D T ) for various substituents and hydrogen production. They found that the D T for catalysts with F-, Cl-, and Br-substituted ligands was 1.768 Dy, 1.975 Dy, and 2.085 Dy, respectively, which does not correspond to the trends observed in HER rates (Fig. 8g ). It was evident that the ligand’s polarity alone is insufficient to determine the catalyst’s reactivity. This was followed by the evaluation of the spatial distribution of relevant orbitals and their interactions in nanoparticle-ligand systems to understand the orbital contributions in electron transfer rates. They observed that the synergy between both the polarities of the ligand and interfacial orbitals interactions affect the electron transfer in the Au-ligand system and, hence, the catalyst’s efficiency. A comprehensive model combining these parameters was proposed, represented by the function D T 2 / J , where J was the charge transfer integral, and a low value of the function corresponded to a system with favorable electron transfer characteristics and high HER activity. A strong correlation was identified between the computed D T 2 / J and the observed reactivity, highlighting the substantial influence of polarity and orbital effects on the catalyst’s efficiency (Fig. 8h ). Despite having a higher D T , the HER rate for the catalyst with a p-Br substituted ligand surpassed that of Cl- and F-substituted catalysts. This disparity was attributed to the elevated value of J for the Br-substituted ligand, facilitating enhanced electron transfer and activity. The synergistic effect of dipole and orbital interactions augmented reactivity for Br-substituted catalysts compared to others. Their findings underscored the feasibility of predicting HER activity for ligand-nanoparticle systems based on the amalgamation of dipole and orbital couplings in the catalyst, encapsulated in the D T 2 / J function (Fig. 8h ).
Furthermore, evaluation in dark conditions demonstrated no hydrogen production, affirming the dominance of the non-thermal pathway in catalysis. This study not only elucidated the involvement of hot electrons but also introduced a predictive function based on dipole and orbital interactions to assess electron transfer efficiency in the catalyst, establishing the critical role of non-thermal effects in HER activity 138 .
Chandra et al. undertook a comprehensive investigation into the utilization of multiple hot holes in plasmonic catalysis, shedding light on an often-overlooked aspect of this field 159 . Their study focused on the plasmon-activated step-down oxidation of styrene to benzaldehyde and formaldehyde in the presence of t-butyl hydrogen peroxide (tBuOOH, TBHP) at room temperature (296 K), facilitated by visible photoexcitation of spherical gold nanoparticles (Au NPs). Synthesized via a seed-mediated approach, the Au NP catalyst exhibited a characteristic LSPR absorption in the visible region at 525 nm, with spherical morphology and an average diameter of 14.5 ± 1.5 nm (Fig. 9a, b ). Under 450 nm light irradiation, the Au NP catalyst selectively oxidized styrene to benzaldehyde with high conversion efficiency (58.0 ± 2.5%) in 10 h, contrasting sharply with the low yield (11.2 ± 2.1%) observed in the dark (Fig. 9c ). They then evaluated the role of light in a light on-off study. They observed a sharp increase in conversion when the light was switched on as compared to the conversion in the dark (Fig. 9 d). Additionally, when the light was switched off, there was a sharp increase in the conversion which decreased with time. This was due to the change in the adsorption equilibrium during light on and off conditions (Fig. 9d ). They further carried out a wavelength-dependent study by choosing three different light wavelengths which were 450 nm (interband excitation), 520 nm (LSPR excitation), and 640 nm (off-resonant excitation) at constant temperature and photon flux, 0.205 W cm −2 . They observed the highest conversion for interband transition, and not for LSPR excitation. This indicated that the electric field enhancement due to LSPR did not contribute significantly (Fig. 9e ). They also carried out control experiments in dark conditions to examine the role of local heat due to LSPR excitation however, the conversion was significantly low in the dark conditions as compared to light conditions which indicated that the local heat (thermal pathway) was not the primary driving force in activation of reactant, molecules by Au NPs. On carrying out the intensity-dependent catalysis, they observed that at lower light intensities (0–165 mW cm −2 ) the conversion followed a linear trend (Fig. 9f ). However, as the intensity increased beyond a threshold intensity of 165 mW cm −2 , a multifold increase in the product conversion was observed i.e., a transition from linear to super-linear which indicated that the reaction was driven by a multiphoton-driven mechanism leading to an increase in hot charge carrier generation, which in turn resulted in multifold enhancement in substrate activation (Fig. 9f ). On doing the kinetics studies they observed a decrease in ΔH ‡ for the light-mediated reaction which gave a clear indication of the reduction in the activation barrier. The large negative value of ΔS ‡ under light illumination indicates the association between alkene and oxygen from t-butyl hydrogen peroxide (TBHP). They concluded that this mechanism involved substrate donation of σ- and π-electrons, followed by cyclic oxometallate species formation with C-C bond scission as the rate-determining step. These findings provided valuable insights into the energetic underpinnings of the reaction mechanism, emphasizing the pivotal role of charge carriers generated from the interband excitation of plasmonic metals, particularly the often-overlooked role of multiple hot holes in substrate activation 159 .
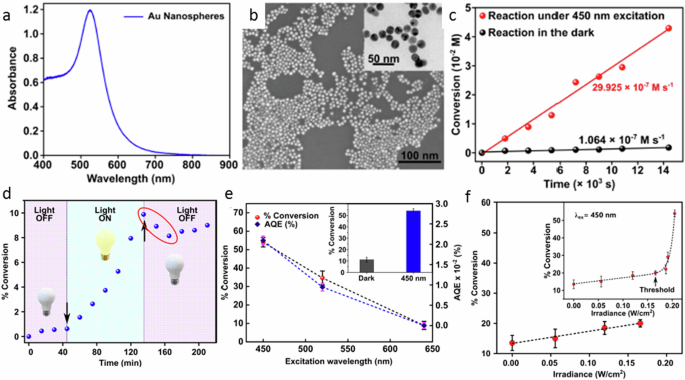
a Absorption spectrum of a spherical Au NP catalyst displaying a characteristic LSPR absorbance at 525 nm, b SEM and TEM images (inset) of the Au NPs with an average size of 14.5 ± 1.5 nm, c Progress of the oxidative cleavage of styrene to benzaldehyde under light-irradiated (at 450 nm with COB-LED) and dark conditions using TBHP as an oxidizing agent, d Monitoring the conversion of styrene to benzaldehyde as a function of time at light-ON ( λ ex = 450 nm, 205 mW cm −2 ) and light-OFF condition, at 293 K. Under light-OFF condition, there was no product conversion; when the light was turned ON, there was an increase in the product formation and upon turning the light OFF again the reaction reversed for a brief period (encircled in red) and thereafter showed limited conversion, e conversion of styrene to benzaldehyde (left axis) and apparent quantum efficiency (AQE, right axis) as a function of excitation wavelength (at constant temperature and photon flux, 0.205 W cm −2 ). The plot shows an increased % conversion and AQE at interband excitation. Inset shows the overall reaction conversion under light illumination (at 450 nm, blue bar) and dark condition (gray bar), f Light intensity dependence in modulating the conversion of styrene to benzaldehyde. The figure is adapted with permission from ref. 159 . Simultaneous harvesting of multiple hot holes via visible-light excitation of plasmonic gold nanospheres for selective oxidative bond scission of olefins to carbonyls. Angew. Chem. Int. Ed . © 2022 Wiley-VCH GmbH.
Plasmonic catalysis via thermal pathways
In this section, we discuss the role of “thermal pathways” in propelling diverse catalytic reactions. This is important for reactions involving gas-solid and gas-liquid interaction because of the temperature gradient present at the interface 254 . The excitation of electrons by light energy perturbs the equilibrium state of electron distribution, generating hot electrons that is generally harnessed for photocatalysis. However, to comprehensively grasp the dynamic behaviors of these hot electrons, it’s crucial to consider the potential scenarios wherein apart from the rise of electronic temperature due to electron-electron collisions, phonon temperature also increases due to electron-phonon interactions, and this thermal energy then transferred to the reactants from the lattice 229 , 230 , 231 , 232 , 233 , 234 , 235 , 236 , 237 , 238 , 239 , 240 , 241 , 242 , 243 , 244 , 245 , 246 , 247 , 248 , 249 , 250 , 251 , 252 , 253 , 254 , 255 , 256 , 257 , 258 , 259 , 260 , 261 , 262 , 263 , 264 , 265 , 266 , 267 , 268 , 269 , 270 , 271 , 272 , 273 , 274 , 275 .
However, the crux of the challenge lies in defining the temperatures of these “hot electrons” due to their departure from equilibrium. Temperature, being inherently an equilibrium property, encounters complexities in its characterization under these conditions. Addressing this issue, Sivan et al. proposed a theory that addresses the photogeneration of non-equilibrium high-energy carriers within metal nanostructures 236 . Their approach involved devising a quantum-like variant of the Boltzmann equation (BE), a tool commonly employed to describe electron dynamics within metallic systems larger than a few nanometers. Through their work, they demonstrated an increase in the population of non-equilibrium high-energy electrons and holes when subjected to illumination. Nevertheless, this process remained highly inefficient, with the majority of absorbed energy contributing to heating. The electron and phonon temperatures exhibited good similarity, thereby justifying the adoption of a classical single-temperature heat model. This theoretical framework initiates with the Boltzmann equation, which serves as the foundation for their approach:
In this equation, f denotes the electron distribution function at an energy ε , electron temperature T e , and phonon temperature T ph . It signifies the likelihood of finding electrons in a system with a continuum of states within the conduction band. To simplify the analysis without compromising precision, the authors opted to disregard any discrepancies that might arise from the phonon system deviating from thermal equilibrium. The essence of the equation can be dissected into three distinct terms, each encapsulating a pivotal process that governs the behavior of the electron distribution (Fig. 10 ).

a Absorption of photons by an electron, with an energy quanta ħω, b Electron-phonon scattering, c Electron-electron scattering, d deviation from the equilibrium distribution at the ambient electron temperature as a function of electron energy for various incoming field levels, e Comparison of the true non-equilibrium distribution Δ f NT ≡ f ( ε , Te, Tph) – f T ( ε , Te) with Δ f within the energy range close to the Fermi energy, f The populations of electrons at E = 1.8 eV above the Fermi level (blue rectangles) and electrons at E = 2.5 eV (> ħω ) above the Fermi level (yellow triangles). The figure is reprinted with permission from ref. 236 , CCBY https://creativecommons.org/licenses/by/4.0/ , copyright 2019 Springer Nature without any changes.
In this study, the authors used relaxation time approximation to describe e–e collisions. In this model, it was assumed that the non-equilibrium electron distribution relaxes to a Fermi-Dirac form f T ( ε , T e ) with a well-defined temperature T e , having the form:
here τ e–e is the is the electron collision time. The electron temperature associated with the Fermi-Dirac distribution represents the temperature at which the electron subsystem would stabilize if the incident light were to cease and no further energy exchange transpired with the phonon subsystem. This approach effectively simplifies the computation of the electron-electron collision integral, highlighting the inherent principle within the comprehensive collision integral framework: the inclination of the electron system to attain a Fermi-Dirac distribution is overtly demonstrated. The crux of the relaxation time approximation lies in its capacity to resolve the ambiguity inherent in determining the electron subsystem’s temperature. Afterward, attention turned to determining T ph , which controls the speed of energy transfer from the electron subsystem to the phonon subsystem and, eventually, to the surroundings. To consistently determine T ph while preserving energy conservation, a perspective that encompassed the system’s “macroscopic” characteristics became essential. To this end, they multiplied Eq. ( 11 ) by the multiple of the electron energy ε and the density of electron states ρ ( ε ), followed by integration across the electron energy spectrum. The resulting equation effectively encapsulated the dynamics associated with the energy of the electrons:
This suggests that the dynamics of electron energy is influenced by how the energy from photoexcitation comes in and how the energy flows out to the lattice. Likewise, the overall energy of the lattice, U ph , was maintained through the exchange of heat from the electronic system coming in and flowing out to the surroundings:
In this equation, T env represents the temperature of the environment, situated at a considerable distance from the nanostructure. G ph−env is directly related to the thermal conductivity of the environment, with a significant dependence on the geometric characteristics of the nanostructure. On finding the steady state solutions of Eqs. ( 11 )–( 14 ), one can get an idea about the temperatures in a system that is out of equilibrium.
To separate the non-thermal component, the authors examined the difference between the electron distribution and the distribution at the steady-state temperature, denoted as Δ f NT ≡ f ( ε , T e , T ph ) – f T ( ε , T e ). Close to the Fermi energy, the population change was roughly an order of magnitude smaller and exhibited an opposite trend (Fig. 10 ). This unexpected outcome suggested that the nonthermal distribution just above (below) the Fermi energy involved the presence of non-thermal holes (electrons). Such findings were only possible by explicitly separating the three energy channels, allowing Te to surpass T env 236 .
For energies beyond ħω from the Fermi energy, the nonthermal distribution was considerably lower, requiring multiple photon absorption. This indicated that for effectively utilizing the excess energy of non-thermal electrons, it is crucial to limit the harvested energy to processes requiring less energy than ħω. The study underscored that most absorbed power induces a shift in the electron distribution near the Fermi energy rather than generating high-energy electrons. It emphasized that any interpretation of experimental results dismissing electron and phonon heating should be approached with caution. Similar insights were gained by adjusting the electron-phonon coupling coefficients and parameters of the Arrhenius equation in various studies involving diverse plasmonic systems, reactions, and photophysics 236 .
A recent study conducted experiments aimed at investigating the solar-driven CO 2 methanation using the Rh/Al nanoantenna catalyst, conducted across various light intensities and chamber temperatures (Fig. 11a–c ) 237 . A notable trend emerged where the production rate of CH 4 experienced a sharp increase upon elevating the light intensity from 8.9 to 11.3 W cm −2 (Fig. 11d ). This phenomenon indicated a photothermal reaction mechanism prevailing over conventional photocatalysis. The CH 4 selectivity remained consistently close to 100% under diverse light intensities. Further exploration into the interaction between assistant heating and photothermal CO 2 methanation led to raising the chamber temperature to 200 °C, which resulted in even greater enhancements in CH 4 production rates which established thermal pathways as the key mode of activation of the CO 2 molecule (Fig. 11e, f ) 237 .
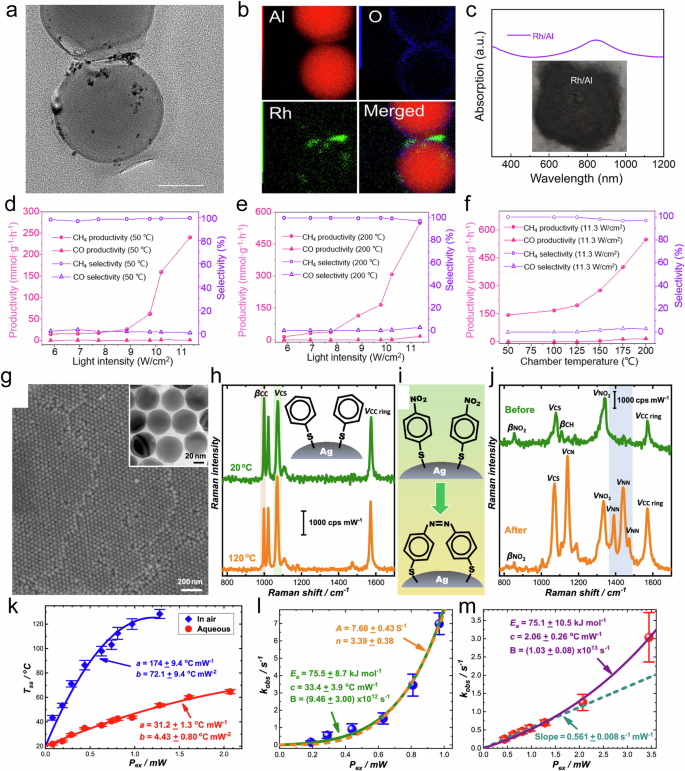
a TEM, scale bar: 50 nm, b corresponding elemental mapping, and c UV–vis absorption spectra of the Rh/Al nanoantenna catalyst, CH 4 and CO productivity and selectivity under different intensities at the chamber temperatures of d 50 and e 200 °C, f Productivity and selectivity at different chamber temperatures, g SEM image of Ag nanoparticle arrays on a Si substrate, h SERS spectra of TP on Ag nanoparticle, i Schematic illustration of the eductive coupling of pNTP, j SERS spectra of pNTP on Ag nanoparticle arrays before and after laser illumination at a P ex of 0.97 mW in the air for 20 s, k P ex -dependence of T ss in air and an aqueous medium. P ex -dependence of k obs pNTP coupling reactions in l air and m an aqueous environment. a – f is reprinted with permission from ref. 237 . Copyright 2021 American Chemical Society. g – m is reprinted with permission from ref. 238 . Copyright 2023 American Chemical Society.
In a recent investigation conducted by Wang et al., they employed SERS as an in situ spectroscopic tool with surface sensitivity to establish connections between intricate kinetic attributes of plasmon-mediated molecular transformations and the localized temperatures at active sites on photocatalyst surfaces 238 . To enable this, they organized spherical silver nanoparticles (diameters of 45 ± 4 nm) covered with monolayers of thiolated molecular adsorbates into hexagonally close-packed arrays on silicon substrates, ensuring that the gaps between particles were kept below 10 nm (Fig. 11g ). The study harnessed SERS to monitor the structural changes occurring in molecular adsorbates within these “hot spots” during both plasmon-induced photothermal heating and photocatalytic reactions. Their SERS-based temperature measurement strategy entailed the use of chemisorbed thiophenol (TP) as a molecular probe, employing SERS characteristics inherently sensitive to temperature variations. After subjecting the samples to thermal treatment at elevated temperatures, the relative SERS intensity of the ring scissoring mode (β CC , 1000 cm⁻¹) underwent a substantial reduction compared to that of the C–S stretching mode ( ν CS , 1072 cm⁻¹). This shift resulted from thermally induced irreversible changes in molecular conformation (Fig. 11h ) 238 .
In the context of photocatalytic reactions, they focused on the plasmon-driven reductive coupling of para-nitrothiophenol (pNTP) on Ag NPs surfaces, yielding the formation of an aromatic azo compound known as p,p′-dimercaptoazobenzene (DMAB) (Fig. 11i ). The transformation from pNTP to DMAB was monitored using SERS due to the distinctive SERS features displayed by these two compounds (Fig. 11j ). The SERS peaks characteristic of pNTP, such as the benzene ring mode (ν CC ring, 1572 cm⁻¹), the nitro stretching mode (ν NO2 , 1334 cm⁻¹), the C–S stretching mode (ν CS , 1072 cm⁻¹), the nitro scissoring mode (β NO2 , 856 cm⁻¹), and the C–H bending mode (β CH , 1105 cm⁻¹), showed changes in their relative intensities following laser illumination. Specifically, the relative intensities of the ν NO2 and β NO2 modes decreased compared to the ν CC ring mode. The authors aimed to shed light on the role of thermal and non-thermal contributions in this reaction.
They introduced an empirical parameter, denoted as “Q,” which quantified the intensity ratio between the β CC mode at 1000 cm⁻¹ and the ν CS mode at 1072 cm⁻¹ within the SERS spectra of TP. They found that Q consistently decreased as the surrounding bulk temperature progressively rose across a wide range from 20 °C up to 120 °C. This enabled them to establish a correlation between Q and the temperature of the catalyst. Further investigations centered on examining the dependency of Q ss ( Q steady state in light) on light power ( P ex ). The temperature T ss was deduced from the value of Q ss , enabling the establishment of the relationship between T ss and P ex . It was observed that Tss increased with P ex , demonstrating a sublinear dependence on P ex . This trend was fitted using a quadratic polynomial function (Fig. 11k ). Typically, under moderate light excitation conditions, a linear relationship between T ss and P ex was observed, given that the contribution of the nonlinear response term was substantially smaller compared to the linear response term. However, at significantly high P ex levels, the nonlinear term’s influence became more pronounced due to various factors, including modifications in material permittivity, shifts in plasmon resonance frequencies, reduced quality factors of plasmonic cavities, and alterations in thermal properties of interfaces and local environments. Even though the excitation powers studied ranged from sub-milliwatt (sub-mW) to milliwatt (mW), the power densities at the focal point reached magnitudes on the order of 10 3 to 10 4 W cm⁻², considerably surpassing those conventionally employed in plasmonic photocatalysis. Thus, the observed sublinear dependence of Tss on P ex in this context was consistent with expectations.
Likewise, superlinear relationships between the observed rate constant ( k obs ) and P ex were observed (Fig. 11l , m). Transitioning the surrounding medium from air to an aqueous solution led to significant decreases in both k obs and Tss. The intricacy introduced by factors such as surface adsorbates, silicon substrates, and interparticle interactions made the mechanisms of heat generation and dissipation complex, especially when contrasted with those of an isolated nanoparticle dispersed within a uniform dielectric medium. Results from the SERS-based temperature and kinetic measurements highlighted that the observed superlinearity in the power dependence of reaction rates within the plasmon-driven bimolecular coupling of pNTP predominantly stems from photothermal heating, rather than nonthermal plasmonic effects. This observation diverged from numerous other studies where similar trends were attributed to the involvement of hot carriers, as opposed to photothermal heating indicating the complex nature of plasmonic catalysis 238 .
Liu et al. explored light-induced thermal gradients in light-assisted ammonia production using a plasmonic photocatalyst much thicker than the light penetration depth 243 . They employed cesium-promoted, magnesium oxide supported, ruthenium (Ru-Cs/MgO) having weak broad plasmon resonance due to Ru NPs, which limited hot carrier generation but facilitated plasmonic photothermal heating (Fig. 12a ). The temperature profile was measured by using two embedded thin thermocouples one at the top-surface ( T 1 ) and the other at the bottom-surface ( T 2 ) (Fig. 12b ). The temperature gradient ( ∇ T = T 2 − T 1 ) was positive in case of dark condition where the energy was provided by an external heater at the bottom of the catalyst bed, whereas in light case it was negative due to the photoheating of the top catalyst surface (Fig. 12c ). It was observed that even at similar equivalent temperature condition the production rate in case light was more than the dark case. The authors attributed this enhancement in light to the strong negative thermal gradient created within the catalyst bed (Fig. 12d ). Even under room temperature and only light illumination conditions, 858 μmol g −1 h −1 of ammonia was formed (Fig. 12e ). The temperature dependence of reaction quotient ( Q P ) and isothermal equilibrium constant ( K iso ) indicated that for Te > 450 °C, Q P > K iso (Fig. 12f ). This was attributed to the illumination augmented gradients within the catalyst which caused significant deviations from the conventional isothermal conditions. This established the role of thermal gradient and thermal effect in the reaction. The gradient and hence, the yield were found to show an exponential dependency on the light intensity (Fig. 12g ). The ammonia production was significantly lower in the case of NIR excitation. This was because the Ru NPs weakly absorb the NIR light and hence the NIR light penetrates deeper into the catalyst bed thereby reducing the gradient and yield (Fig. 12g ). On doing the wavelength-dependent studies at two equivalent temperatures, it was established that not only does Te determined reaction rates, the size and sign of ∇ T were also important (Fig. 12h, i ). In this study, authors not only established the role of thermal effects but also showed how these effects can be tailored by improving the catalyst light illumination, intensity, and wavelengths to tune the thermal gradient present within the catalyst bed to get the desired output 243 .
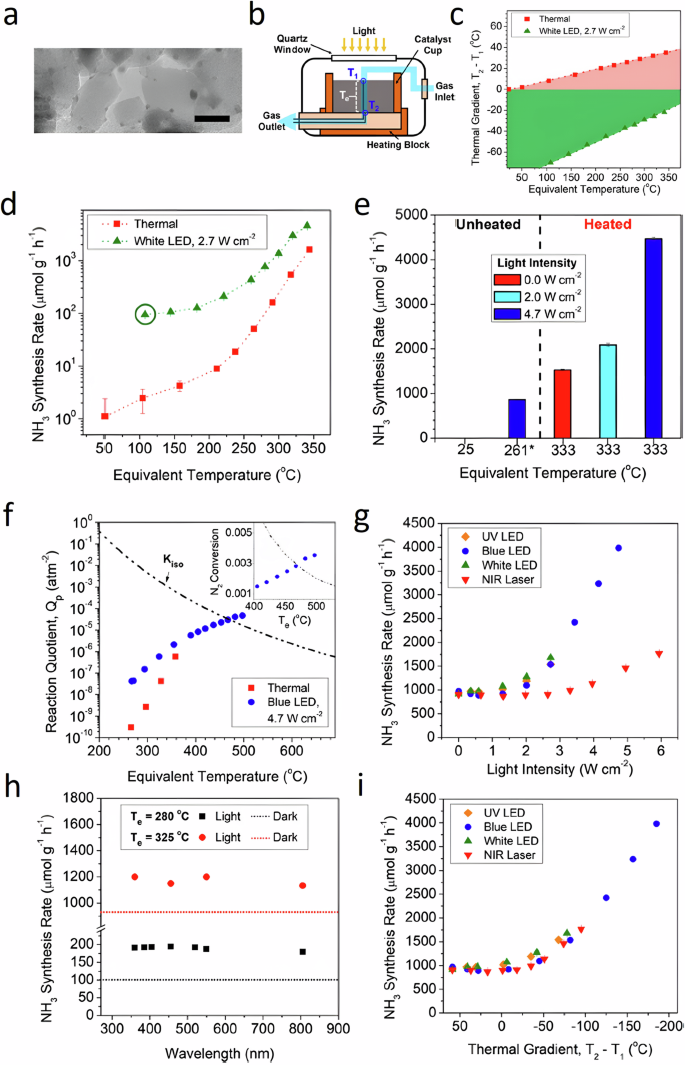
a TEM image of the Ru–Cs/MgO catalyst, scale bar: 20 nm, b Schematic representation of reaction chamber for in situ measurements of top- ( T 1 ) and bottom- ( T 2 ) surface temperatures of the catalyst bed, c Measured thermal gradients under dark thermal and heated white light illumination, d Measured dark thermal and heated white light illumination NH 3 synthesis rates as a function of equivalent temperature. Circled data point indicates the light-only condition with no external heating applied, e NH 3 synthesis rates under dark and additionally illuminated conditions using a blue LED, f Experimental reaction quotient, Q P , for dark heated, combined light and heat conditions, and calculated isothermal equilibrium constant, K iso , as a function of equivalent temperature, Te, g Measured NH 3 synthesis rates as a function of light intensity for Te = 325 °C using UV, blue, and white LEDs and the NIR laser, h Measured wavelength-dependent ammonia synthesis rates ( ∇ T < 0) with Te set at 280 °C and 325 °C, compared to dark thermal rates, at similar light intensities for both Te at a particular wavelength [for Te = 280 °C, 365 nm (2.2 W cm −2 ), 385 nm (2.2 W cm −2 ), 405 nm (2.2 W cm −2 ), 455 nm (2.1 W cm −2 ), 525 nm (2.1 W cm −2 ), 550 nm (2.2 W cm −2 ), 805 nm (4.5 W cm −2 ); for Te = 325 °C, 365 nm (2.0 W cm −2 ), 455 nm (1.9 W cm −2 ), 550 nm (2.0 W cm −2 ), 805 nm (4.1 W cm −2 )], i Measured NH 3 synthesis rates as a function of the thermal gradient for Te = 325 °C. The figure is reprinted with permission from ref. 243 . Copyright 2019 American Chemical Society.
In another interesting investigation, Rodríguez-López et al. employed scanning electrochemical microscopy (SECM) in the feedback and H 2 O 2 collection modes to understand the effects of plasmonic excitation 256 .The investigation was carried out on graphene-covered gold nanoparticle arrays (G-AuNP) which served as a template to directly compare subsurface effects on the interfacial reactivity. The sample was made up of 50 μm wide arrays, containing a well-confined monolayer of ∼ 50 nm AuNPs (Fig. 13a, b ). To understand the effect of plasmon excitation, oxygen reduction reaction (ORR) was studied at the G-AuNP electrode. The substrate was biased to reduce oxygen and the tip was biased to collect the generated H 2 O 2 . Under these conditions, any changes in the current sensed at the probe were assigned to arise from changes in the mass transport. A change in ORR kinetics was observed in the case of light illumination. This was validated by the observation of a lower overpotential to activate the generation of H 2 O 2 . This was experimentally observed as a positive shift in the i-E curve for H 2 O 2 collection (Fig. 13c ). On performing the substrate generation tip collection (SGTC) CVs, the collection curves followed the trend consistent with an increase in the standard rate constant (k o ) and mass transfer (Fig. 13d ). To further investigate this aspect, Au NPs were covered on a 10-layer graphene (10L-G-AuNP). However, in both the cases of G-AuNP and 10L-G-AuNP, the natural logarithm of the calculated k o ″ showed a positive response to increasing the laser power (Fig. 13e, f ). This followed the Arrhenius behavior for temperature-dependent kinetics suggesting the involvement of photothermal effects was the main contributor to enhanced electrochemical reactivity. This study highlighted a very important point that even in liquid phase systems thermal effects can play a pivotal role and they can’t be ignored based on the argument of thermal gradient and high heat transport due to convection and conduction. It is all about the kinetics and time scales associated with the reaction and heat transport. If the kinetics of the reaction is fast then the thermal effect may play a pivotal role in enhancing the rate of the reaction 256 .
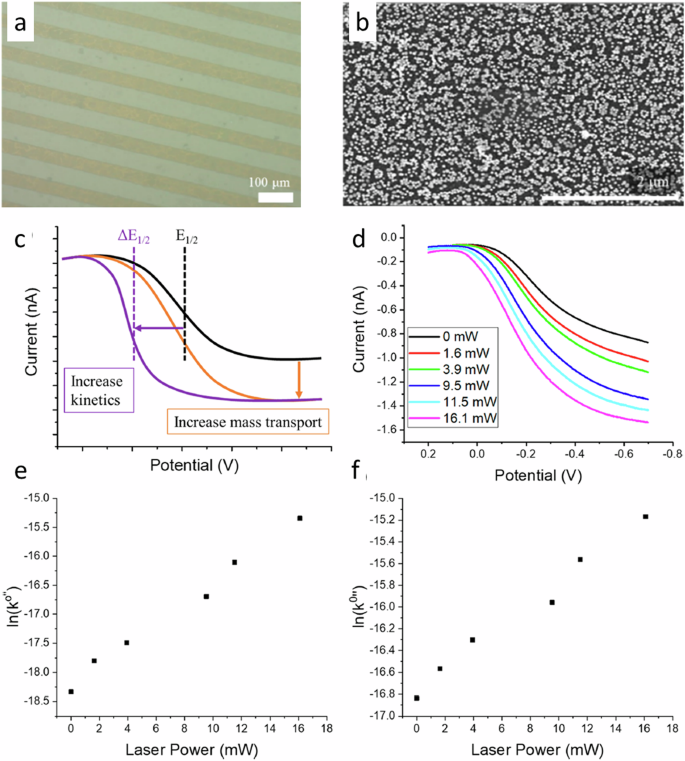
a Optical image of G-AuNP, b SEM images of G-AuNP. Collection of H 2 O 2 at the tip during ORR at the substrate, c Representation of collection data showing the expected response to an increase in diffusion versus an increase in kinetics, d Tip current during substrate ORR CV at increasing laser powers, e The plot of calculated ko″ of ORR at the G-AuNP substrate with increasing laser power, f The corresponding increase in ko″ of ORR at the 10-G-AuNP interface with laser power. The figure is reprinted with permission from ref. 256 . Copyright 2020 American Chemical Society.
In their comprehensive study of thermochromism, Pillai et al. leveraged plasmonic heating of an array of gold nanorods aiming to quantify practically usable thermoplasmonic heat near the surface of nanostructures 257 . Their research encompassed photothermal transformations conducted both in solution (aqueous and organic) and in solid states. Thermoplasmonic properties of gold nanorod (AuNR) arrays were effectively demonstrated across various applications, including thermally driven polymerization, solar-vapor generation, Diels–Alder reaction, and crystal-to-crystal transformation processes (Fig. 14a ).
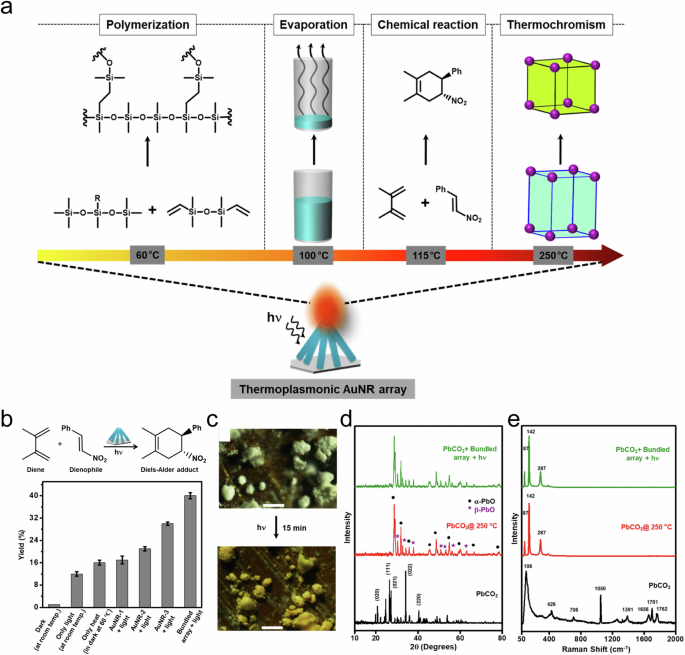
a Use of thermoplasmonic AuNR arrays for performing photothermally driven pdms polymerization, solar-vapor generation, Diels–Alder [4 + 2] cycloaddition, and crystal-to-crystal transformation processes. Thermoplasmonic Diels–Alder reaction, b Reaction scheme for the [4 + 2] cycloaddition between 2,3-dimethyl-1,3-butadiene (diene) and trans-β-nitrostyrene (dienophile), using the thermoplasmonic heat generated from bundled AuNR arrays; Bar diagram summarizing the yield of the Diels–Alder adduct obtained under different reaction conditions. Quantification of thermoplasmonic heat through thermochromism, c Optical microscopic images showing the color changes in PbCO 3 upon irradiation in the presence of bundled AuNR arrays. The scale bar corresponds to 200 μm. The corresponding d PXRD and e Raman spectra confirm the transformation of orthorhombic PbCO 3 to a mixture of α- and β-lead oxide (tetragonal and orthorhombic phases). The figure is reprinted with permission from ref. 257 . Copyright 2022 American Chemical Society.
In their exploration of thermally driven reactions, they focused on the atom-economic and widely employed Diels−Alder cycloaddition in synthetic organic chemistry. Employing a [4 + 2] cycloaddition between 2,3-dimethyl-1,3-butadiene and trans-β-nitrostyrene, solely heat-driven, they achieved a notable 40% yield of the Diels−Alder adduct (1S,2R)-4,5-dimethyl-2-nitro-1,2,3,6-tetrahydro-1,1’-biphenyl within 15 h under the irradiation of a 1 W green laser (Fig. 14b ). The absence of products corresponding to the photochemical [2 + 2] cycloaddition further underscored the role of light-induced heating in the observed reaction. Their investigations also discussed the utilization of thermochromism to monitor the surface temperature of bundled AuNR arrays, leveraging the visual color change as a readout (Fig. 14c ). Lead carbonate (PbCO 3 ) served as the chosen thermochromic molecule, undergoing a phase transformation into a mixture of α- and β-lead oxide (PbO) at approximately 250 °C, accompanied by a distinct color change from white to yellow. Detailed powder X-ray diffraction (PXRD) and Raman studies provided unequivocal evidence of the quantitative photothermal transformation of PbCO 3 to PbO upon laser irradiation (Fig. 14d, e ). Their findings, coupled with conventional thermal quantification techniques, revealed that temperatures close to the surface of AuNR arrays could reach up to approximately 250 °C within approximately 15 min of laser irradiation. Moreover, direct IR thermometric imaging elucidated crucial insights into heat generation and dissipative pathways. Additionally, they highlighted the significant influence of reaction conditions on heat dissipation from the thermoplasmonic surface to the surroundings, with minimal observed impact on surface temperature in solution-state studies. This study shed light on the multifaceted applications of thermoplasmonic heat, underscoring its potential in diverse fields 257 .
Plasmonic catalysis via synergy, competition, and detrimental effect of thermal and non-thermal pathways
In this section, we discussed examples of catalytic reactions where both thermal and non-thermal pathways were observed for the same catalyst, and a synergy between both pathways was established 74 , 185 , 230 , 233 , 235 , 276 , 277 . Liu et al. in one of their works on CO 2 methanation on Rh/TiO 2 catalysts tried to estimate the contribution of thermal and non-thermal pathways by using an indirect illumination technique in conjunction with precisely monitored thermal profiles of the catalyst 230 . They excited the catalyst by using direct and indirect illumination techniques and the thermal profile of the catalyst was monitored using a multi-thermocouple setup, and an equivalent temperature (Te) was calculated from the measured central temperatures of the top ( T 1 ) and bottom ( T 2 ) surface (Fig. 15a ). The indirect illumination was achieved by placing a photothermal material that is inactive for the reaction on the Rh/TiO 2 photocatalyst. This helped to maintain the same thermal profile of the catalyst in the dark and light conditions. The indirect illumination prevented the nonthermal plasmonic effects, such as hot carrier generation or enhanced local fields because in this case, the top Ti 2 O 3 powder was acting as a photothermal heater to achieve the same T 1 , T 2 , and temperature profile within the Rh/TiO 2 photocatalyst as would occur without the Ti 2 O 3 overlayer. In the case of a heated Rh/TiO 2 photocatalyst subjected to direct light irradiation, a dynamic interplay between heat and light significantly influenced the CH 4 production rates, particularly within a temperature range Te < ∼ 350 °C (Fig. 15b ). This interaction manifested in an enhancement of CH 4 production rates, indicative of the synergistic effects of heat and light. However, as temperatures exceed this threshold, a divergence emerged wherein the measured total CH 4 production rate ( R tot ) diminishes compared to analogous dark thermal conditions at equivalent Te. This phenomenon stemmed from the intricate balance between kinetic and thermodynamic factors inherent in exothermic reversible reactions such as CO 2 methanation. The optimal temperature for such reactions represents a compromise point between kinetic and thermodynamic considerations. Initially, CH 4 production rates exhibit exponential growth with increasing operating temperatures. However, at elevated temperatures, the favorable conditions for the reverse reaction of CH 4 reforming begin to prevail, offsetting the forward reaction kinetics (Fig. 15b ). Furthermore, the authors observed that at Te > ∼ 350 °C, the temperature of the catalyst ( T 1 ) can surpass the temperature of the external environment ( T 2 ) even under dark conditions due to the exothermic nature of the reaction (Fig. 15b ). The self-heating propensity of the catalyst resulted in a nearly isothermal thermal profile, rendering the calculated thermal rate ( R t,c ) unreliable under high-intensity illumination, where non-isothermal conditions were amplified 230 . Subsequent experimentation, involving the maintenance of the top surface at a constant temperature T 1 while manipulating light intensity and external heating facilitated the monitoring of total CH 4 production rates and thermal profiles as functions of light intensity (Iuv) and T 2 (Fig. 15c–f ). Under direct illumination, the measured total methane production rate of Rh/TiO 2 exhibited a characteristic “U” shape due to evolving light intensities and temperatures. At lower light intensities, maximal external heating is necessitated ( T 2 > T 1 ), and the reaction predominantly assumed a thermal nature. However, with increasing Iuv, the requirement for external heating diminishes, and T 2 approaches T 1 , which results in a decrease in the reaction rate. Moreover, as light intensities escalated, and the temperature gradient ( ∇ T ) changed significantly, the catalyst surface became the hottest region, leading to the dominance of photothermal and nonthermal effects, thereby amplifying R tot . The intricacies of this phenomenon became apparent, where the shape of the “U” was influenced by T 1 , while nonthermal effects became significant for Iuv > 0.5 W cm −2 for all T 1 (Fig. 15c–f ). Moreover, the utilization of various LEDs and light intensities in indirect illumination scenarios enabled the replication of thermal profiles, thereby unveiling the thermal component of the R tot rates observed under direct illumination. Since Ti 2 O 3 serves solely as a light absorber and was inert in CO 2 methanation, the measured CH 4 production rates remain independent of light wavelength. Despite potential challenges in accurately calculating thermal contributions at high intensities where gradients were intensified, the nonthermal light contribution can be deduced by subtracting the measured thermal contribution from R tot . This extracted rate, albeit potentially containing a blend of effects from nonthermal hot carriers and superheated NPs, was largely mitigated by the negligible temperature increase induced by plasmonic heating of metal NPs above their environment, especially on supports with high thermal conductivity. Under direct illumination, any temperature increase in Rh NPs quickly equilibrated with the temperature of the TiO 2 support, thus rendering the measured T 1 a reasonable representation of the surface temperature. Furthermore, investigations into purely photothermal systems reveal that the measured total production rate and corresponding thermal contribution remain consistent under both direct and indirect illumination, underscoring the robustness of the experimental approach in capturing the thermal contribution from light 230 .
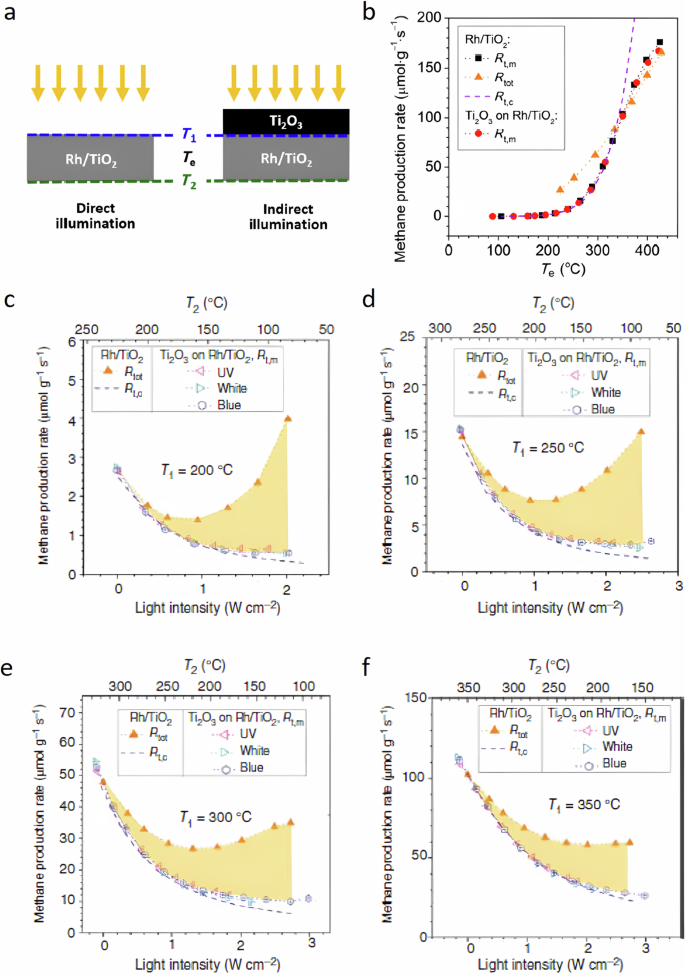
a Schematic representation of photocatalytic reactions under direct and indirect illumination, b CH 4 production rates are shown as a function of Te. For the uncovered Rh/TiO 2 catalyst, the measured rate under dark thermal conditions ( R t,m , black squares) and under direct illumination of 2.73 W cm −2 UV light with additional external heating ( R tot , orange triangles) are plotted. For the covered catalyst, the measured rate under dark thermal conditions ( R t,m , red circles) is shown. The calculated thermal CH 4 production rate ( R t,c , purple dashed lines) as a function of Te is also shown in the figure. CO 2 methanation using direct vs. indirect illumination. For top surface temperature T 1 = ( c ) 200, ( d ) 250, ( e ) 300, and ( f ) 350 °C, the CH 4 production rates are plotted as a function of UV light intensity and corresponding bottom surface ( T 2 ) temperature. Under direct illumination, measured total rate R tot (orange triangles) and calculated R t,c (purple dashed lines) are shown. Under indirect illumination, measured CH 4 production rates, R t,m , are plotted using UV (pink left triangles), white (green right triangles), and blue (navy hexagons) LEDs. The yellow-shaded region represents the nonthermal contribution. The figure is reprinted with permission from ref. 230 , CCBY https://creativecommons.org/licenses/by/4.0/ , copyright 2019 Springer Nature without any changes.
In an innovative investigation led by Zheng et al., the synergistic interplay between thermal and non-thermal effects was explored in the oxidation reaction of inorganic nanomaterials, coupled with the optimization of crystal structures to elucidate the plasmonic catalysis effect 233 . The study aimed to quantitatively assess the contribution of oxidative activity of hot electrons and thermal effects for LSPR with the assistance of plasmonic gold nanoparticles (Au NPs) (Fig. 16a ).
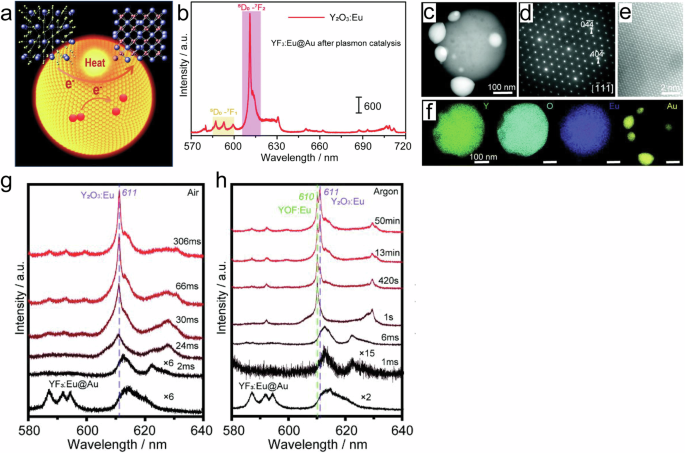
a Sketch of hot electron and thermal effects in plasmon catalytic crystal transformation from YF 3 to Y 2 O 3 . Characterization of the product particle after plasmon catalytic nanocrystal transformation from YF 3 :Eu 3+ to Y 2 O 3 :Eu 3+ , b Luminescence spectra for the product, c HAADF-STEM image, d SAED pattern, e atomic resolution HAADF-STEM image, and f EDX elemental mapping of the product. Evolution of the luminescence spectra for plasmon catalytic transformation under 1 ms laser irradiation (20 mW at the sample). g YF 3 :Eu 3+ @Au in air, and h YF 3 :Eu 3+ @Au in argon. The figure is used with permission of H. Zheng, from ref. 233 ; permission conveyed through Copyright Clearance Center, Inc.
The researchers utilized a YF 3 :Eu 3+ @Au composite submicron structure, synthesized via a coprecipitation procedure, to investigate the plasmonic catalysis of materials. Upon irradiation with a continuous-wave (CW) laser at 532 nm, a rapid plasmon assisted material transformation was observed, resulting in enhanced luminescence intensity and monochromaticity. Characterization techniques including energy dispersive X-ray (EDX) elemental mapping and selected area electron diffraction (SAED) confirmed the transformation from YF 3 to single crystal Y 2 O 3 with a cubic structure (Fig. 16b–f ). Additionally, changes in morphology from bundle-like sub microstructures to spherical nanoparticles were observed, accompanied by the growth of Au NPs on the surface. Further examination revealed that thermal effects predominantly drove the plasmon-catalyzed material transformation, as evidenced by experiments involving conventional thermal catalysis. Annealing of YF 3 particles resulted in gradual transformation to Y 2 O 3 , with the crystal structure and luminescence spectra indicating successful oxidation. Moreover, investigations under different atmospheres demonstrated distinct transformation rates and product compositions, highlighting the influence of oxygen availability on the oxidation process (Fig. 16g, h ). The role of hot electrons in catalyzing material transformation was explored through in situ monitoring of plasmon catalytic reactions under different conditions. The transfer of hot electrons to surface molecules, particularly oxygen, facilitated oxidation reactions by generating superoxide anions with strong oxidation potential. The catalytic effect of hot electrons activated reactants and intermediate species, lowering activation energies and thereby enhancing reaction rates. Overall, this study underscores the significant contributions of both thermal and non-thermal effects, particularly hot electron catalysis, in driving material oxidation and crystal transformation processes 233 .
Our lab has extensively investigated the intricacies of plasmonic catalysis utilizing a material known as black gold across a spectrum of reactions, including CO 2 hydrogenation, acetylene semi-hydrogenation, and C-Cl bond activation 74 , 96 , 185 , 235 . Black gold, also known as dendritic plasmonic colloidosomes (DPC) or black Au, was synthesized by loading gold nanoparticles onto dendritic fibrous nanosilica (DFNS) using a meticulously controlled cycle-by-cycle approach (Fig. 17a ) 74 . This methodology enabled precise manipulation of nanoparticle sizes and interparticle distances, facilitating efficient plasmonic coupling and inducing a shift in optical properties, transitioning the material’s color from red to black. This transformation enabled the material to absorb light across the entire visible and near-infrared regions of the solar spectrum (Fig. 17b ).

a Cycle-by-cycle growth approach for dendritic plasmonic colloidosomes with varying Au particle sizes and interparticle distances, b Absorption spectrum of DPC-Cx with various numbers of growth cycles, c Photocatalytic CO 2 conversion to methane using DPC-C1, DPC-C3, DPC-C4, and DPC-C6 as catalysts under light. Plasmonic CO 2 hydrogenation using Black gold-Ni. d Production rate of CH 4 and CO in light at various intensities and in the dark at different temperatures. No external heating was used in the case of light experiments, e KIE for CO 2 hydrogenation, measured in dark and light. Black gold–Ni catalyzed hydrodechlorination of DCM to methane in flow f at different light intensities, g in the dark at various temperatures, h KIE for hydrodechlorination of DCM, measured in light and dark. Acetylene semi-hydrogenation in the presence of excess ethene using DPC/RuPt, ethene, and ethane production rate i at different light intensities, and j in the dark at various temperatures, k KIE for acetylene semi-hydrogenation, measured in light and dark. a – c are reprinted with permission from ref. 74 , CCBY https://creativecommons.org/licenses/by/4.0/ , copyright 2019 Royal Society of Chemistry without any changes, d and e are reprinted with permission from ref. 96 . Copyright 2023 American Chemical Society. f – h is reprinted with permission from ref. 235 . Copyright 2023 American Chemical Society. i – k is reprinted with permission from ref. 185 , CCBY https://creativecommons.org/licenses/by/4.0/ , copyright 2024 Springer Nature without any changes.
In our initial investigations, black gold exhibited photocatalytic activity for CO 2 reduction under light irradiation, albeit with relatively modest product yields (Fig. 17c ). We attributed this to factors such as the short lifetime of hot electrons or the weak affinity of Au sites for CO 2 molecules 74 . To address these limitations, we engineered a catalyst, termed black gold-Ni or DPC-Ni, by incorporating nickel nanoparticles onto the black gold substrate 96 . This modification aimed to enhance CO 2 activation on Ni sites and prolong the lifetime of hot electrons. Encouragingly, DPC-Ni demonstrated excellent activity for the CO 2 hydrogenation reaction under light irradiation in a flow reactor, with CO productivity increasing with light intensity (Fig. 17d ). Surface temperature monitoring during dark conditions revealed negligible CO productivity, suggesting a potential hot electron-assisted mechanism (Fig. 17d ). Further evidence supporting the involvement of hot electrons was obtained through kinetic isotope effect (KIE) measurements, showing an increased KIE value under light irradiation compared to dark conditions (Fig. 17e ) 96 .
Subsequent investigations into the hydrodechlorination of dichloromethane using DPC-Ni revealed a notable synergy between thermal and non-thermal pathways 235 . Methane productivity and catalyst surface temperature exhibited a positive correlation with light intensity (Fig. 17f ). Interestingly, while negligible productivity was observed in the dark at lower temperatures, a sharp increase occurred beyond a threshold temperature of 170 °C, with methane production matching light-induced rates at 245 °C (Fig. 17g ). KIE measurements further supported this observation, demonstrating a less pronounced increase in KIE values under light compared to CO 2 hydrogenation even for the same catalyst, indicative of the combined influence of both thermal and non-thermal activation pathways (Fig. 17h ) 235 . These examples highlight that the contribution of thermal and non-thermal pathways can change even for the same catalyst if the reaction is altered.
In a recent study, we explored the catalytic capabilities of black gold loaded with RuPt NPs (DPC-RuPt) for the photocatalytic semi-hydrogenation of acetylene 185 . Ethene productivity and catalyst surface temperature exhibited a positive correlation with increasing light intensity (Fig. 17i ). Similarly, in dark conditions, ethene productivity increased with temperature, with rates matching those achieved under light at 220 °C (Fig. 17j ). Consistent with previous observations, KIE measurements highlighted the role of non-thermal activation under light, although control experiments in dark conditions underscored the significant synergy between thermal and non-thermal pathways (Fig. 17k ) 185 .These findings underscore the complex interplay between thermal and non-thermal pathways in plasmonic catalysis, emphasizing the importance of factors such as reaction type, catalyst composition, and light intensity in determining catalytic outcomes.
The thermal and non-thermal effects can also compete with each other instead of working in synergy. This sometimes results in changes in product selectivity, kinetics parameters, reaction intermediates, etc. 84 , 278 . Li et al. investigated a competitive effect in formic acid (FA) dehydrogenation using Pd-based plasmonic catalysts, observing a kinetic switch from a competitive Langmuir-Hinshelwood (L-H) adsorption mode in the dark to a non-competitive mode under irradiation 278 . This switch was driven by local field effects and hot carriers. The authors designed a series of Pd-based hybrid plasmonic nanostructures with precisely controlled geometric and electronic properties to study the adsorption behavior of reactants during plasmon excitation.
Using a seed-mediated overgrowth method, they synthesized various Pd-based plasmonic hybrid catalysts, including Au@Pd, Au@AuPd, and Au@AgPd core-shell nanoparticles, ensuring similar absorption spectra across the nanostructures (Fig. 18a ). The thickness of the shell was precisely controlled to get similar absorption of three nanostructures (Fig. 18b ). The catalysts’ performance was assessed based on H 2 production rates normalized to Pd mass. Under irradiation, all samples exhibited higher activities compared to their dark conditions at the same temperature, indicating light-enhanced H 2 evolution. Au@AgPd demonstrated superior catalytic activity, followed by Au@AuPd, and then Au@Pd. The study further explored the underlying kinetics of FA dehydrogenation (Fig. 18 c–f). In the dark, the hydrogen production rate as a function of reactant concentration followed a typical competitive L-H mechanism, where the rate initially increased and then decreased with higher reactant concentrations due to competitive adsorption of HCOOH and HCOO − on the same surface sites (Fig. 18c ). Under illumination, however, the mechanism shifted to a non-competitive L-H type, with the H 2 evolution rate increasing and then plateauing at saturation, indicating non-competitive adsorption behavior (Fig. 18d ). This mechanism switch was observed across all catalyst types.

a Schematic illustration of the preparation of three nanostructures, and b UV–vis extinction spectra of various samples in an aqueous solution, c , d The H 2 evolution rates on Au@Pd, Au@AuPd, and Au@AgPd in the dark and under irradiation (25 °C, 200 mW cm −2 ) in the presence of different FA and SF concentrations with a fixed FA/SF proportion of 1:1. The H 2 evolution rates on Au@AgPd with e different SF concentrations under a fixed FA concentration (1 M), and f different FA concentrations without SF, g Schematic of the plasmon-switched kinetics from a competitive mode in the dark to a non-competitive mode under irradiation. The figure is reprinted with permission from ref. 278 . ChemSusChem © 2024 Wiley-VCH GmbH.
Fixing the FA concentration to 1 M revealed similar competitive adsorption in the dark and non-competitive L-H behavior under light irradiation (Fig. 18e ). In the absence of sodium formate (SF), no gaseous hydrogen was detected, underscoring the negligible role of formate hydrolysis. The hydrogen generation rate significantly dropped without SF compared to an FA/SF system of the same concentration (Fig. 18f ). Without SF, no competitive adsorption was observed in the dark, with a positively linear correlation between activity and FA concentration, which became super-linear under irradiation (Fig. 18f ).
These findings indicated that catalytic activity depends on formate concentration within a specific limit where significant adsorption competition was absent. Excess SF increased partial pressure after adsorption equilibrium, occupying more sites and causing dehydrogenation decay. This competitive behavior was common in thermal catalysis, but in an aqueous environment, a competitive adsorption mode shifts to a non-competitive one upon visible light irradiation on a plasmonic photocatalyst (Fig. 18g ). DFT calculations revealed that electron sites in all facets predominantly favor HCOOH adsorption compared to hole and neutral sites, while hole sites preferentially adsorb HCOO − . This plasmon-induced selective adsorption behavior aligns with the non-competitive mode under irradiation, altering the overall reaction kinetics between light and dark conditions. This study underscores the competitive nature of thermal and non-thermal pathways in plasmonic catalysis 278 .
The thermal and non-thermal effects can also interact detrimentally, suppressing each other’s effectiveness 279 , 280 , 281 , 282 . Lyutakov et al. reported one such inverse temperature effect on the reaction rate in plasmon-assisted click chemistry, revealing a detrimental interplay between thermal and non-thermal effects 279 . In conventional plasmonic catalysis, the process involves several key steps: (1) plasmon excitation of organic molecules via external electron injection or HOMO-LUMO intramolecular electron transition, (2) vibrational relaxation of molecules in the excited state, and (3) relaxation of injected or excited electrons, resulting in an “activated” molecule with a lower residual barrier for the desired chemical transformation. This residual barrier is typically overcome by conventional heating. Thus, under normal circumstances, light and temperature synergistically facilitate the reaction-light excites plasmons, activating nearby molecules, while heat helps overcome the residual thermodynamic barrier. However, in scenarios where the residual barrier is eliminated, temperature can adversely affect the reaction rate. Higher temperatures may increase electron-phonon scattering, reducing plasmon lifetime and the probability of reactant excitation. Additionally, elevated temperatures could shorten the lifetimes of electronically excited molecules, making the desired reaction less likely 279 .To investigate this phenomenon, the authors studied the azide-alkyne cycloaddition (AAC) reaction under plasmon-assisted conditions. They grafted the alkyne reagent onto the surface of spherical gold nanoparticles (AuNPs) using a diazonium approach with 4-ethynylphenyl groups (Fig. 19a–c ), positioning these chemical moieties close to the plasmonic evanescent wave. The successful immobilization of 4-ethynylphenyl units was confirmed by Raman spectroscopy (Fig. 19d ), while TEM analysis verified that the AuNPs retained their morphology and size post-grafting (Fig. 19c ). The grafted AuNPs in acetonitrile exhibited a distinct plasmon absorption band near 650 nm in the UV–Vis spectrum (Fig. 19e ). The reaction was carried out at −35 °C and the activity decreased with an increase in the reaction temperature. DFT calculations were conducted to explore potential AAC pathways, focusing on the reactivity of differently activated phenylacetylene (Fig. 19f ). Two primary modes of plasmonic activation were considered: hot electron injection from metal nanoparticles and intramolecular HOMO-LUMO electron excitation. The calculated energies for various possible reaction pathways suggested that while hot-electron injection cannot be excluded, the dominant mechanism appears to be pathway PW1 (Fig. 19f ). In conclusion, the observed inverse temperature dependence of the reaction rate was attributed to decreased electron-phonon scattering at lower temperature and delayed relaxation of organic molecules following plasmon-induced excitation. This study demonstrated a scenario where thermal effects can suppress non-thermal plasmonic effects in a catalytic reaction, highlighting the complex interplay between these mechanisms 279 .

a Schematic representation of the used experimental concept–grafting of the AuNP surface with 4-ethynylphenyl groups and the subsequent plasmon-induced click reaction on the AuNP surface, b proposed principle of the decrease of the AAC residual activation energy, c TEM images of AuNPs before and after grafting with 4-ethynylphenyl groups, d SERS spectra of pristine AuNPs and AuNPs–C≡CH, e UV–Vis spectra of AuNPs–C≡CH in acetonitrile and the emission spectrum of the LED source further used for plasmon excitation, f DFT-calculated (B3LYP-D3/6-311++G(d,p)) reaction pathways under plasmon assistance and related transition states. E 0 ’ values indicate calculated redox potentials vs. Fc/Fc + . The figure is used with permission of O. Lyutakov, from ref. 279 ; permission conveyed through Copyright Clearance Center, Inc.
Ultrafast dynamics in plasmonic catalysis
The complexity of plasmon-assisted catalysis is amplified by the nanoscale size of NPs and the short lifetimes associated with LSPR 1 , 4 , 9 , 283 , 284 . To investigate the dynamics of plasmons, researchers have increasingly utilized ultrafast tools to decipher these rapid processes. As discussed in the “Plasmon-mediated chemical reactions” section of this review, chemical reactions facilitated by plasmonic catalysis can theoretically follow various pathways, including near-field enhancement, charge transfer, or local temperature increase. The specific pathway depends on multiple factors, such as the nature of the metal NPs, the particular reaction and reactants involved, and environmental parameters like dielectric properties, refractive index, band gaps, and electron mobility. Several studies have explored these aspects on the ultrafast time scale, shedding light on the intricate mechanisms at play 285 , 286 , 287 , 288 , 289 , 290 .
Kuisma et al. conducted a detailed investigation into the real-time dynamics of plasmon formation and dephasing into hot carriers (HCs) using comprehensive first-principles calculations 285 . They quantitatively analyzed the impact of atomic-scale structure on HC generation, modeling an icosahedral Ag 561 silver NP as a representative system with a clear plasmon resonance in the photoabsorption spectrum (Fig. 20a ). The ground-state electronic structure of the NP was calculated using DFT with the Gritsenko–van Leeuwen–van Lenthe–Baerends–solid-correlation (GLLB-sc) exchange-correlation (XC) potential to improve the description of the d-band. For the time-dependent response, time-dependent DFT (TDDFT) calculations were performed using either the random-phase approximation (RPA) or the adiabatic GLLB-sc. A monochromatic ultrafast Gaussian light pulse was employed to excite the LSPR, inducing real-time electron dynamics within the system. The pulse frequency ( ω 0 = 3.6 eV) was tuned to the plasmon resonance, with a pulse duration ( τ 0 ) of 3 fs, centered at t 0 = 10 fs (Fig. 20b ). The light pulse created a time-dependent external potential, causing the time evolution of the Kohn-Sham (KS) states and excitation of the LSPR. This induced a strong dipole-moment response (Fig. 20c , panels 1–3). The resulting electron density oscillations (Fig. 20d , panels 1–3) comprised a surface-to-surface component associated with delocalized valence electrons near the Fermi energy and atom-localized contributions corresponding to screening due to virtual excitations from the d-band. As time progressed to t ≈ 17 fs, the excited electrons began losing their collective plasmonic motion through a dephasing process known as Landau damping. This dephasing was evidenced by the decay of the dipole moment (Fig. 20c , panels 4, 5) and the disappearance of surface-to-surface density oscillations (Fig. 20d , panels 4, 5).

a Photoabsorption spectrum of the Ag 561 NP (shaded) and the intensity profile of an impacting electric field pulse (green), b Electric field pulse impacting the plasmon resonance of the NP, c Time-dependent dipole moment response of the NP, d Electron density oscillations in the NP at selected time instances (red and blue isosurfaces denote density increase and decrease, respectively), e Time evolution of the energy stored in the excited electronic system. The total energy (black), nonresonant electron–hole transition (purple), resonant transition (orange), and Coulomb energy (gray). Electron–hole transition contributions to plasmon formation and decay. f Electron–hole contributions to the photoabsorption at the resonance energy visualized as a transition contribution map (TCM). Density of states is also shown along the energy axes, g Electron-hole contributions to the time-dependent electronic energy are visualized as TCM on a logarithmic color scale. The solid diagonal line corresponds to the transition energies matching with the pulse frequency ω 0 and the dotted diagonal lines are drawn at ω 0 ± 2 σ to indicate the pulse width σ = 2/ τ 0 , defining the boundaries for resonant and nonresonant transitions for Fig. 20e. h Occupation probabilities of hole and electron states. Solid blue and red lines denote state occupations from resonant transitions, and dashed lines denote occupations from all transitions (resonant and nonresonant). The figure is reprinted with permission from ref. 285 , CCBY https://creativecommons.org/licenses/by/4.0/ , copyright 2020 American Chemical Society without any changes.
As the pulse was tuned to the LSPR, the electronic system absorbed energy from the incident light and remained excited after the pulse had dissipated. To analyze the energy distribution, the authors considered the total time-dependent energy of the system, given by:
where E (0) tot is the ground-state energy, Δ E ( t ) is the time-dependent energy stored in the excited state (Fig. 20e , black line), and E pulse ( t ) = − μ ( t ) ε ( t ) is the potential energy of the system under the external electric field. The incident light pulse pumps energy into the system in a stepwise manner (Fig. 20e , t ≈ 5…15 fs). Although the total energy remained constant, the electronic energy was not evenly distributed among the electron−hole transitions excited by the light pulse. To quantify this effect, the authors decomposed the energy into electron-hole transition contributions. Plasmon formation and dephasing were scrutinized by considering the energy stored in the electronic system in terms of the electron–hole transition energy relative to the pulse frequency. It was found that the plasmon was formed by the constructive coupling of low-energy transitions ( ≲ 2 eV) (Fig. 20f and time instances (1–3) in Fig. 20g, h ). Simultaneously, high-energy virtual d-electron transitions ( ≳ 4 eV) screened the plasmonic density oscillation, reducing the total induced field. Nonresonant low- and high-energy transitions carried most of the energy during plasmon excitation (Fig. 20e , purple line). As the plasmon dephased, the absorbed energy was redistributed to electron–hole transitions resonant with the pulse (Fig. 20e , orange line; corresponding to the diagonal in the transition contribution maps in Fig. 20g, h ; time instances (4, 5)). After dephasing, the energy remained almost exclusively in these transitions, constituting the plasmon-generated HCs. This was a crucial finding as it clearly distinguished between the types of excitation (resonant and non-resonant) carrying plasmon energy.
However, the study did not discuss the dynamics at longer time scales after the energy finally dissipated to the environment as local heat, radiation, or other processes. The coupling of transitions via Coulomb interaction was recognized as an essential characteristic of plasmonic excitations, making it important to consider the Coulomb energy. This energy exhibited strong oscillations (Fig. 20e , gray line) analogous to the dipole moment (Fig. 20c ), as only the electron density oscillation contributed to the Coulomb energy 285 .
The authors further analyzed the distribution of plasmon-generated HCs and the impact of local atomic-scale structure by considering a series of icosahedral silver NPs Ag 147 , Ag 309 , and Ag 561 . They tuned the light pulse to the plasmon resonance of the NPs and examined the initial HC distributions after the plasmon had been dephased at t = 30 fs. The HC distributions exhibited a pronounced dependence on NP size (Fig. 21a ) and local structure (Fig. 21b, c ). As particle size increased, the HC distributions were increasingly dominated by interband d-electron transitions (hole ∼ −4 eV → electron ∼ 0 eV), converging towards the distributions obtained for flat surfaces. Due to the broken crystal symmetry, additional intraband transitions were available for plasmonic HC generation in NPs, resulting in the population of higher-energy electron and hole states (Fig. 21a ; electron states of >0.5 eV, hole states of >−3.5 eV). The relative contribution of these sp-states was most pronounced in the smallest NPs (Ag 147 , Ag 309 ), but they remained significant in Ag 561 .
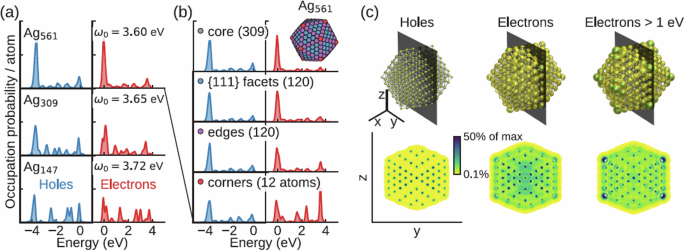
a Occupation probabilities of hole and electron states in icosahedral silver NPs of 147–561 atoms. b Occupation probabilities at different atomic sites of Ag 561 . c Spatial density profiles of all induced holes and electrons and induced electrons with energy of more than 1 eV in Ag 561 . The figure is reprinted with permission from ref. 285 , CCBY https://creativecommons.org/licenses/by/4.0/ , copyright 2020 American Chemical Society without any changes.
The calculated probability distributions of plasmon-generated electrons and holes exhibited strong spatial variance for the icosahedral Ag 561 NP (Fig. 21b, c ). Holes were localized at atomic sites throughout the particle, as most originated from the atom-localized d-states, resulting in a similar energy distribution for core and surface sites. In contrast, hot electrons were more delocalized and predominantly resided in the surface region. The surface contribution was even more pronounced for higher-energy (>1 eV) hot electrons (Fig. 21c ). The authors found that on silver NPs, lower-coordinated surface sites exhibit a larger proportion of hot electrons, especially those with higher energy, than the bulk of the nanoparticle or higher-coordinated surface sites. In contrast, the distribution of hot holes is relatively homogeneous within each considered NP. This study highlighted the significant role of NP size and local atomic structure in determining the time scale, distribution, and energy of plasmon-generated HCs, emphasizing the importance of surface sites for efficient HC utilization in plasmonic catalysis 285 .
Nagpal et al. also investigated the role of various scattering mechanisms—electron-electron, electron-phonon, impurity, surface, and grain boundary scattering—on the decay of LSPR 283 . To prevent surface oxidation, they applied smooth conformal atomic layer deposition (ALD) coatings of alumina on copper nanoparticles. This allowed them to study the dephasing of LSPR in different-sized copper nanoparticles. Through quantitative analysis and various temperature-dependent measurements, they observed that electron-phonon interactions predominantly contributed to the dephasing of plasmon waves, overshadowing other scattering mechanisms. Although interband transitions in Cu metal significantly contributed to plasmon losses, tuning surface plasmon modes to infrared frequencies resulted in a five-fold enhancement in the quality factor. This study underscored the importance of electron-phonon interactions in the dephasing process and highlighted the potential for enhancing plasmonic quality factors by adjusting the size and shape of NPs 283 .
In the previous examples, we discussed how dephasing creates high-energy carriers and the associated time scales. The next step involves utilizing these excited charge carriers and understanding the related time scales. Sá et al. investigated the time scales associated with hydrated electrons generated by the excitation of copper LSPR 287 . The authors used transient absorption spectroscopy (TAS) to detect the hydrated electrons and understand the dynamics involved. Hydrated electrons were observed upon excitation at the LSPR absorption maximum, to induce hot electrons to possess sufficient energy to be ejected from the Cu NPs. The photo-redox capabilities of the hydrated electrons were confirmed in a catalytic proton reduction reaction, leading to the evolution of molecular hydrogen on adjacent Ru/TiO 2 NP species. The synthesized Cu NPs were molecularly grafted onto TiO 2 anatase with β-alanine, featuring an LSPR peak centered around 395 nm (Fig. 22a, b ). Ultrafast TAS was used to evaluate the formation of hydrated electrons. The excitation wavelength was varied across the Cu NPs LSPR, and the photoinduced changes were probed between 350 and 800 nm. In the case of NPs, LSPR excitation led to spectral broadening of the surface plasmon absorption, resulting in transient bleaching at the center of the plasmon band maximum and two positive absorption wings at lower and higher energies in the difference spectrum. The transient signal showed the expected “bleaching” of the LSPR absorption band and a winglet band centered at 480 nm (Fig. 22c ). Analysis of the transient behavior of the winglet signal provided information about copper plasmonic relaxation dynamics (Fig. 22d ). The kinetic trace extracted at 500 nm was fitted with a rising component and three-exponential decay functions. The rising component was estimated to be around 400 fs and was assigned to electron–electron scattering. The kinetic trace was fitted with two major exponential decay curves with characteristic lifetimes of 4 and 45 ps and a smaller long-lived component (>2 ns). The fastest component was ascribed to electron–phonon scattering, while the 45 ps decay feature related to phonon–phonon relaxation.

Characterization of Cu NPs used in this study. a representative HRTEM micrograph of Cu NPs on TiO 2 anatase, b UV–visible spectrum of Cu NPs after synthesis, Ultrafast TAS analysis of Cu NPs, c two-dimensional color map of the temporal evolution of the surface plasmon resonance of Cu excited at 398 nm, d kinetic trace extracted at 500 nm depicting the signal decay of positive absorption induced by plasmon excitation (“winglets”), e kinetic trace extracted at 700 nm depicting the signal ascribed to hydrated electrons, with black dashed line illustrating the time regime where prehydrated and hydrated electrons coexist; after 10 ps, the signal can be associated with only hydrated electrons, f normalized signal intensity at 690–710 nm as a function of excitation wavelength at two distinct times, namely, 0.5 (prehydrated + hydrated electrons) and 100 ps (hydrated electrons only), and g laser power dependence of the hydrated electron and winglet signals (intensities extracted at 100 ps), h hydrogen evolution profile as a function of reaction time upon CW laser excitation at 405 nm, and i methylene blue photo-oxidation conversion profile reaction time upon CW laser excitation at 405 nm (red trace) Cu NPs linked with β-alanine to TiO 2 with Ru NPs and (black trace) blank experiment with all components present except Cu NPs. The figure is reprinted with permission from ref. 287 . Copyright 2019 American Chemical Society.
Careful analysis of the two-dimensional color map (Fig. 22c ) revealed a long-lived broad absorption signal starting at 600 nm with a maximum around ~700–720 nm, which was assigned to solvated electrons. Analysis of the kinetic trace extracted at 700 nm revealed two distinct regions: a fast-decaying component (3 ps) accounting for roughly 70% of the signal and a long-lived component that barely decayed over the experimental delay line (5 ns) (Fig. 22d ). The faster component was assigned to prehydrated electrons on the Cu NP surface, while the long-lived component was associated with hydrated electrons (Fig. 22e ). The hottest electrons, formed by resonant excitation of the LSPR, carried sufficient energy to transfer into a hydrated state. Consequently, one should expect a decrease in hydrated electron formation when the excitation wavelength is detuned from the Cu LSPR maximum. The signal intensity in the 690–710 nm region as a function of excitation wavelength (390–450 nm) at two distinct times: at 0.5 ps (prehydrated + hydrated electrons) and 100 ps (hydrated electrons only) (Fig. 22f ). Indeed, once the excitation wavelength deviated from the LSPR maximum, the population of prehydrated and hydrated electrons rapidly decreased nearly to zero, confirming that only the LSPR excitation in Cu NPs can yield electrons with sufficient energy to create hydrated electrons. Further evidence that the hydrated electrons were due to the excitation of the Cu NPs LSPR and subsequent hot carrier generation was found in the signal laser power dependence (Fig. 22g ). The quadratic behavior of the hydrated electron signal intensity versus the laser power contrasts with the linear dependence for the winglet intensity versus the laser power. Superlinear power dependencies of rates and intensities are characteristic of plasmon-induced hot carrier processes. The hot carrier distribution was strongly affected by electron–electron scattering, with an average energy that increases with incident power when the time interval between subsequent photons becomes smaller than the lifetimes of the hot carriers.
To explore the possible use of hydrated electrons in photocatalysis, the authors conducted an experiment involving plasmonic hot electron transfer. They examined the dynamics of excited electrons by changing the molecular linker from β-alanine to p-aminobenzoic acid, a conductive molecular linker with a similar length. They observed a significantly larger signal and faster injection when β-alanine was replaced by p-aminobenzoic acid. H 2 evolution from Ru NPs and methylene blue oxidation on Cu NPs showed that the injected hydrated electrons catalyzed proton reduction on the Ru NPs (Fig. 22h, i ). The holes left behind in the Cu NPs were refilled through methylene blue oxidation, prolonging H 2 evolution beyond 20 min (Fig. 22i ). In this work, the authors effectively utilized the power of TAS to extract the dynamics of hot carriers in Cu NPs. The detection of hydrated electrons clearly showed that hot electrons had sufficient energy to transfer from Cu NPs to the surrounding environment to become hydrated electrons, which were then utilized for H 2 production. This demonstrated that plasmon decay results in higher-energy (hot) electrons and (cold) holes, which can be harnessed for practical applications in photocatalysis 287 .
Ultrafast tools also help us understand the dynamics of the thermal effects of LSPR. Alivisatos et al. employed time-resolved infrared spectroscopy in their investigation to monitor alterations in the vibrational mode of local water occurring over hundreds of picoseconds 288 . The investigative technique relied upon the shifts in infrared combination absorption bands of water, known for their sensitivity to temperature variations. Through the utilization of time-resolved infrared (TRIR) spectra of water, alterations in infrared absorption were leveraged to track the flow of heat into the solvent from the gold nanorod (GNR). The authors inspected the combination band encompassing the H–O–H bend and libration modes ranging from 1960 to 2210 cm −1 . Initially, the IR was calibrated as a thermometer using steady-state heating. The TRIR spectra sum IR signals from an ensemble of locally heated solvent molecules surrounding numerous particles within the probe area, facilitated by the high temporal resolution of the technique, enabling the estimation of average dynamics of an ensemble of many single particles (Fig. 23a, b ). Following the photoexcitation of CTAB-capped GNR, the TRIR spectra resembled those observed at elevated steady-state temperatures, indicative of rapid heat transfer from the GNR to the solvent over a few hundred picoseconds (Fig. 23c ). Furthermore, a transient response of the system on a sub-picosecond scale was observed, attributed to the vibrational modes of water during the electron solvation process. Kinetic traces within this spectral region were subjected to a single exponential function fitting to extract heat transfer time constants (Fig. 23d ). The impact of the initial temperature of irradiated NPs on their heat transfer dynamics was elucidated through experiments conducted at various excitation powers (Fig. 23d ). The authors observed that at 2, 4, or 8 mW, heat transfer times remained consistent within experimental error margins, around 350 ps. Conversely, at higher powers such as 20 mW, a discernible acceleration in heat transfer was noted, suggesting the tunability of heat transfer rates with increased excitation power.

a Scheme of laser pulses overlapped in the region of 200 μm in a flow cell, b The probe TRIR signal in ( a ) is attributed to the sum signal of water surrounding locally each NP in the probe region. c , e Spectra at various excitation powers and delay times; d , f , g Kinetic traces of the 2140–2180 cm −1 region of the CTAB capped, thin and thick silica-coated GNR solutions, respectively, show a initial fast decay in tens of picoseconds and a later slow decay in nanoseconds. Fitting time constants (95% confidence) at later delay times (30–3000 ps) at various laser powers. The insets show representative fast decay of signals at early delay times (1–30 ps). Temperature values on the left of each kinetic trace show the estimated highest temperature of the NPs based on the photon fluences. The figure is reprinted with permission from ref. 288 . Copyright 2016 American Chemical Society.
Additionally, the authors explored silica-coated GNRs to discern the effect of silica thickness on heat diffusion rates. Two distinct silica-coated GNR samples, featuring silica thicknesses of approximately 30 nm (thin-coated) and 90 nm (thick-coated), were prepared to evaluate this influence on heat transfer dynamics. The TRIR spectra of the thin-coated GNR samples closely mirrored the difference spectra at elevated steady-state temperatures, indicating a fast decay of positive ΔOD (optical density) signals followed by sustained, although insignificant changes in large positive ΔOD values up to 30 ps (Fig. 23e ). Comparatively, the silica-coated samples exhibited slower heat transfer dynamics in contrast to their surfactant-capped counterparts (Fig. 23f, g ). Moreover, mesoporous silica-coated GNRs demonstrated even slower heat transfer dynamics than CTAB-capped GNRs. The heat transfer time for GNRs coated with a 30 nm thick silica shell was approximately 600 ps, attributable to the partial diffusion of heat from the silica shell to water via the thermally resistant CTAB at the silica shell–water interface. Conversely, in samples featuring a thicker silica coating (90 nm), the heat transfer time was approximately 400 ps owing to efficient heat diffusion within the silica shell. This comprehensive investigation provided valuable insights into the range of heat transfer times and lengths critical for informing the design of optimal shell thicknesses in photothermal applications involving porous silica-coated nanoparticles 288 .
The debate of thermal vs. non-thermal effects
The heart of the matter in the debate between thermal and non-thermal modes of activation resides in a dichotomy of viewpoints, pitting two opposing schools of thought against each other. A noteworthy concern regarding primary hot carriers pertains to their exceptionally low lifetimes, a mere fraction of a picosecond. These carriers swiftly undergo thermalization via electron-electron scattering, rendering their interaction with the surrounding environment exceedingly improbable. This characteristic underscores the complexity of harnessing their potential for practical applications. The average number of primary hot electrons generated in a single nanoparticle over time under illumination can be computed using the following equation:
in this equation, σ abs is the absorption cross-section, I is the power per unit area, τ e–e is the electron–electron scattering lifetime, and hν is the photon energy 11 . When subjected to continuous wave (CW) illumination, the process of thermalization for a hot carrier often concludes before the arrival of the subsequent photon, leading to a reduced count of excited carriers available for instigating chemical transformations. Conversely, with pulse excitation, the count of hot carriers can increase, with a portion of these carriers remaining accessible to partake in chemical reactions. Conversely, the thermal pathway confronts challenges tied to the precise spatial and temporal measurement of the localized temperature elevation surrounding the plasmonic nanoparticle.
An essential distinction arises when considering the excited charge carrier’s lifespan within a small nanoparticle. In this context, the duration of excited electrons is prolonged. This phenomenon is attributed to heightened confinement, an elevated density of states at a granular level, and diminished interactions among electrons 11 . Furthermore, the equilibration period with the lattice is extended due to reduced electron-phonon coupling. This intricate interplay culminates in the creation of negative-ion states within adsorbed or proximal molecules 11 . These negative-ion states, in turn, become responsible for subsequent chemical transformations. A notable instance of this was documented by Halas et al., who revealed that H 2 dissociation on Au nanoparticles could be achieved under light excitation without resorting to external heating 75 . The involvement of hot electrons in this catalytic process was established through a combination of experiments conducted in the absence of light, as well as investigations exploring wavelength-dependent and light intensity-dependent catalysis. DFT calculations further supported these findings 75 .
Numerous other research groups have similarly reported instances of catalysis mediated by hot electrons. These instances encompass a range of reactions, including the reduction of ferric (Fe 3+ ) ions by Au nanoparticles, CO 2 reduction by Au nanoparticles, water splitting, propylene epoxidation, dry reforming, oxygen dissociation, and more 58 , 63 , 66 , 80 , 81 , 96 , 291 . Each of these studies aimed to illuminate the role of hot electrons through diverse methodologies, including investigations into dark reactions at varying temperatures, catalysis dependent on the light intensity, catalysis contingent on wavelength, analysis of KIE, extraction of thermal and non-thermal contributions through activation energy calculations, ultrafast transient spectroscopy, and more. These experimental endeavors collectively strive to untangle the intricate threads of the non-thermal pathway and establish its pivotal role as a critical activation mechanism in the realm of plasmonic catalysis. A significant challenge emerges within these aforementioned reports, particularly concerning the accurate assessment of the local surface temperature. Various studies, led by researchers such as Sivan et al. and Dubi et al. 236 , 246 , 247 , talked about the role of the thermal pathway within plasmonic catalysis. Central to this perspective is the assertion that the actual local temperature near the nanoparticle can attain exceptionally high values, often surpassing estimations derived from commonly employed techniques like IR cameras and surface-touching thermocouples. These conventional measurement methods, while widely utilized, fall short in terms of spatial resolution required to accurately capture the temperature at the nanoparticle’s surface.
In contrast to relying solely on IR cameras or thermocouples, a distinct approach has been proposed by Sivan et al. 236 . As discussed in the earlier sections this method entails the utilization of coupled Boltzmann-heat equations grounded in principles of energy conservation and fundamental thermodynamics. This derived temperature using this model, generally exceeding those registered by IR cameras or thermocouples, serves as a foundation for calculating activation energies and reaction rates. According to this model, the alteration in electron distribution around the Fermi energy rather than the generation of high-energy electrons significantly diminishes the likelihood of executing chemical transformations. Consequently, the prevailing sentiment underscores the prominence of the thermal pathway as the predominant pathway behind plasmonic catalysis. Some examples of discussions between these two opposing schools of thought are discussed below.
Halas et al. conducted a study involving a Cu-Ru catalyst supported on MgO to produce H 2 from NH 3 81 . Their findings demonstrated a reduction in the activation energy barrier under light exposure, highlighting the substantial influence of “hot” electrons on the reaction, surpassing the impact of purely thermal effects. However, Sivan et al. expressed doubts regarding the interpretation of this report, raising concerns about the measurement of surface temperature via IR cameras and the utilization of intensity and wavelength-dependent activation energies 241 . Sivan et al. employed a light-independent activation energy assumption and postulated a linear correlation between temperature and light intensity to replicate the original results. In their view, the dominant contribution stemmed from the thermal pathway. Halas et al. responded to Sivan et al.’ concern with a comprehensive reply that encompassed several key points 292 :
Justification for temperature measurement: Halas et al. defended their temperature measurement approach by referencing appropriate literature sources that substantiated the chosen emissivity value.
Nonlinearity of temperature increase: the assumption that the temperature of the catalyst linearly increases with light intensity over a broader temperature range was critiqued by Halas et al. This linear model, they argued, is valid only for relatively minor temperature elevations (e.g., around 100 K), and its applicability diminishes for more substantial temperature changes.
Role of activation energy: Halas et al. contended that using a light-independent activation energy is not physically plausible. They noted that the presence of hot carriers introduces changes in adsorbate coverage on the catalyst’s surface, subsequently impacting the apparent activation barrier.
Moreover, the stability of the catalyst at temperatures projected by Sivan et al. was deliberated. Predicted temperatures reached as high as 1150 K, raising concerns about potential issues like melting, aggregation, and sintering of metal nanoparticles. However, no such occurrences were observed in the study by Halas et al., suggesting the possibility of an overestimation of local temperature via the method proposed by Sivan et al. 292 .
This line of questioning concerning light-dependent activation energies, thermal rate extraction, and temperature measurement was also evident in a separate report where Sivan et al. analyzed another study by Halas et al. 73 , 293 . In their response, Halas et al. clarified how the reaction rate was calculated and asserted that Sivan et al. had misinterpreted certain aspects 294 . Photothermal simulations were also conducted to support the accuracy of thermal cameras for measuring the catalyst’s surface temperature. This discourse finally served to establish the presence and significance of hot-carrier contributions to catalytic activity 294 .
Sivan et al., in their report, undertook a comprehensive data analysis of research conducted by Halas and Linic, among others. Their investigation aimed to shed light on the thermal aspects of the reported findings 295 . A central assertion made in this work was that the entire dataset from the original reports by Halas, Linic, and others could be understood through the lens of the thermal pathway. The primary concern highlighted by Sivan et al. revolved around the potential underestimation of local temperature. This was attributed to several factors, including the collective macroscopic heating effect stemming from the presence of numerous nanoparticles in the catalysis experiments, a phenomenon significantly amplifying the minor heating exerted by a solitary nanoparticle. Moreover, potential sources of error were identified, including inaccuracies in employing emissivity values for materials and the presence of temperature gradients within the catalyst due to non-uniform illumination. Sivan et al. published an article where they talked about the distribution of heat under light illumination in these systems 236 . The shifted Arrhenius equation, which was used by them is as follows:
This equation was corrected for illumination-induced heating and was used by Sivan et al. to fit the data from the articles by Halas et al., and Linic et al. 295 . However, a compelling contradiction to these arguments was presented in an article by Jain et al. 296 . In this comprehensive study, Jain et al. started to carefully study the matter, commencing with the assumption that a reduction in activation energy shows a linear relationship with light intensity in photocatalysis. This assumption provided the basis for a thorough investigation into the intricate interplay between light-induced effects and catalytic behavior:
In this equation, B is a proportionality constant with units of eV cm 2 W −1 if E a is expressed in units of eV and I in units of W cm −2 . B is a wavelength-dependent quantity. On further solving the above and putting this in Arrhenius equation, we get:
b is \(\frac{B}{{E}_{a}^{{dark}}}\,\) and has units of cm 2 W −1 .
After using a Taylor expansion around I = 0, which is a dark condition, we get:
Higher-order terms can be ignored in the light-intensity regime ( I < 1/ b ). After putting this in Eq. ( 19 ), we get:
When comparing this equation to the standard Arrhenius equation, it became apparent that the reaction seemed to occur at a theoretical temperature proportional to the light intensity ( I ), which was higher than the actual temperature ( T s ):
This equation was identical to the one employed by Sivan et al. in their work to strengthen their argument for the thermal pathway over the non-thermal pathway. Under the mathematical framework of this equation, the inference drawn is that light excitation solely results in temperature elevation without invoking any alteration in activation energy. This mathematical handling of the Arrhenius equation effectively conceals the potential non-thermal effects of light excitation, camouflaging them as an increase in temperature, i.e., attributing them to the thermal pathway 296 .
This debate over the thermal and non-thermal effects in plasmonic catalysis is still a big question in front of researchers even after so much literature supporting both sides. The exact measurement of local temperature which poses both temporal and spatial challenges is the bottleneck to solve this puzzle. However, there are still indirect signatures and evidence in the catalysis that can be used to get an idea about the exact mechanism which is discussed in the next section.
Experiments to distinguish thermal and non-thermal pathways
In this section, we discuss some of the simple catalysis experimental procedures to understand the relative role of thermal and non-thermal effects in catalysis.
Light intensity dependence of reaction rates
The rate ( η ) of chemical transformation is directly linked to the incident light power on the sample. This correlation holds for CW illumination at moderate light power, and under high power or femtosecond-pulsed laser illumination, it may demonstrate a super-linear trend due to multiphoton absorption 63 , 93 , 95 , 234 . However, a photothermal process behaves differently and does not exhibit such a linear correlation. The temperature increase resulting from light absorption is approximately proportional to the light power incident on the sample. On the other hand, the rate constant ( K ) of a chemical reaction typically follows an Arrhenius-type temperature dependency. Consequently, in a photothermal process, the rate of chemical transformation follows an exponential dependence on illumination power. Prudent care must be taken when employing this methodology as it necessitates the exploration of a wide spectrum of reaction rates 232 , 236 . In this case, precaution is required because, under CW light excitation, the temperature of the catalyst may vary linearly and show a small increase in value. In this small temperature range, the Arrhenius equation may take a linear form and the interpretation may be misled into a completely different direction. This entails utilizing a diverse array of laser powers to prevent any potential ambiguity arising from the distinction between exponential and linear patterns within the limited data points. This happens because any exponential plot when looked at in a small range of variables, will appear linear, but in reality, the system could be still on an exponential path.
Catalyst surface temperature measurements
When the reactive surface is accessible for IR camera imaging, IR thermal measurements are effective for tracking temperature changes 239 , 240 . However, accurate calibration is vital due to black body radiation dependency on temperature and material emissivity. For plasmonic samples, low metal emissivity (~0.1) challenges IR measurements 81 , 241 . Direct thermocouple measurements assess photothermal effects and temperature-depth profiles, but challenges include preventing direct light exposure to the thermocouple, potential substrate-thermocouple contact effects, and ensuring close, effective contact for accurate temperature readings. Temperature microscopy techniques can also be employed, like Raman Thermometry, which involves monitoring the ratio of Stokes and Anti-Stokes lines to back-calculate the catalyst surface temperature (where the probe molecule is adsorbed) 21 , 238 , 242 .
Light-triggered reaction dynamics
When illuminating a macroscopic sample, the time for temperature to stabilize is typically seconds to minutes, governed by thermal diffusivity 69 . This difference in time scales can differentiate photothermal and photochemical effects: rapid rate increase suggests pure photochemistry, while gradual change implies photothermal influence. A converse approach involves turning off the light and measuring reaction rate decay. Nevertheless, these measurements demand an accurate assessment of chemical sensor response time i.e., a high temporal resolution 69 . The temperature measurement using a thermocouple or IR camera will always give the equilibrated temperature and not the local temperature which decays in a few ps to few ns.
Light-wavelength dependent reaction rates
It is feasible to differentiate between thermal and non-thermal pathways through wavelength-dependent investigations at constant light intensity. In such studies, the selection of two distinct wavelengths is imperative—let’s designate them as λ blue (representing high energy) and λ red (indicating low energy). The pivotal requirement for conducting such a study lies in the catalyst manifesting entirely distinct photo processes at these two wavelengths. Let’s assume that λ blue can initiate the formation of hot carriers whereas λ red due to its less energy cannot account for any hot electrons and can only induce thermal effects. If say, blue light enhances the chemical rate more than red light under identical sample temperature conditions, then heating alone cannot account for the photochemical rate increase, indicating the significance of photon energy 63 , 94 , 96 , 235 , 244 . By observing the reaction rate as a function of optical power η ( P ) under two irradiation wavelengths, if distinct behaviors emerge, they are a sign of non-thermal pathways. For thermally driven processes in both cases, there should be a constant factor α that exists, for which η blue ( α . P blue ) = η red ( P red ) with overlapping plots 232 . The constant factor α accounts for the difference in the absorbance at two wavelengths. Conversely, if non-thermal effects activate with λ blue , no such α exists to fulfill the above overlapping conditions. Collecting a rate spectrum could unveil a wavelength threshold where a sharp transition occurs, indicating the quantum nature of excitation as seen in hot-carrier mechanisms. This contrasts with smooth plasmonic resonance absorption seen in photothermal processes. A similar polarization study can also be performed in lithographically aligned plasmonic structures where the chemical reaction rates are found to change with the polarization angle owing to the dependence of near-field effects on polarization 232 , 297 .
Kinetic isotope effect measurements
Kinetic isotope effects offer an alternative method, demonstrating changes in reaction rates when replacing an atom in a reactant molecule with its isotope 63 , 94 , 96 , 99 , 235 , 298 . A higher KIE under light than in the dark at the same temperature indicates the prevalence of non-thermal effects. In the dark, the molecule is vibrationally excited from the ground state by providing external heat. The heavier molecule will have a higher vibrational energy gap and will only get excited to a lower vibrational energy level., thus, a higher value than 1 is expected for KIE. Under plasmonic excitation, reactions occur via transient negative ion formation, inducing electronic excitation in the reactant molecule. The excited molecule navigates the potential energy surface of the excited electronic state, returning to its ground state vibrational energy. The shorter lifetime of the lighter molecule in the excited state, attributed to its greater acceleration and reduced energy loss, leads to higher vibrational energy upon returning to the ground state electronic state. This vibrational energy discrepancy between isotopes after decay results in divergent reaction rates and elevated KIE values under light conditions 63 , 94 , 96 , 99 , 235 , 298 .
Competitive electron transfer reactions
The hot electron transfer mechanism can be validated by conducting the reaction with an electron quencher (e.g., Methyl-p-benzoquinone for CO 2 Hydrogenation) and performing a one-electron reduction reaction (ferrocyanide to ferricyanide) under light exposure 94 , 96 , 235 , 299 . The absence or reduction in catalytic activity in the presence of the electron quencher (Methyl-p-benzoquinone) and a progressive increase in the spectral peak of the reduced species (ferrocyanide) signify the operation of the hot electron mechanism. This study also has a limitation; in this type of study, the adsorption kinetics of the reactant molecule and the electron quencher are assumed to be similar or lie within the same range. However, if the electron quencher has better adsorption, then a decrease in productivity may arise because of the blocking of the active site.
Acknowledgements
We acknowledge the funding support of the Department of Atomic Energy project no. 12-R&D-TFR-RTI4003.
Author information
Authors and affiliations.
Department of Chemical Sciences, Tata Institute of Fundamental Research, Mumbai, 400005, India
Rishi Verma, Gunjan Sharma & Vivek Polshettiwar
You can also search for this author in PubMed Google Scholar
Contributions
V.P. proposed the idea and guided the project. R.V., G.S., and V.P. wrote the article together.
Corresponding author
Correspondence to Vivek Polshettiwar .
Ethics declarations
Competing interests.
The authors declare no competing interests.
Peer review
Peer review information.
Nature Communications thanks Jacinto Sa and the other, anonymous, reviewer(s) for their contribution to the peer review of this work. A peer review file is available.
Additional information
Publisher’s note Springer Nature remains neutral with regard to jurisdictional claims in published maps and institutional affiliations.
Rights and permissions
Open Access This article is licensed under a Creative Commons Attribution-NonCommercial-NoDerivatives 4.0 International License, which permits any non-commercial use, sharing, distribution and reproduction in any medium or format, as long as you give appropriate credit to the original author(s) and the source, provide a link to the Creative Commons licence, and indicate if you modified the licensed material. You do not have permission under this licence to share adapted material derived from this article or parts of it. The images or other third party material in this article are included in the article’s Creative Commons licence, unless indicated otherwise in a credit line to the material. If material is not included in the article’s Creative Commons licence and your intended use is not permitted by statutory regulation or exceeds the permitted use, you will need to obtain permission directly from the copyright holder. To view a copy of this licence, visit http://creativecommons.org/licenses/by-nc-nd/4.0/ .
Reprints and permissions
About this article
Cite this article.
Verma, R., Sharma, G. & Polshettiwar, V. The paradox of thermal vs. non-thermal effects in plasmonic photocatalysis. Nat Commun 15 , 7974 (2024). https://doi.org/10.1038/s41467-024-51916-3
Download citation
Received : 29 February 2024
Accepted : 16 August 2024
Published : 12 September 2024
DOI : https://doi.org/10.1038/s41467-024-51916-3
Share this article
Anyone you share the following link with will be able to read this content:
Sorry, a shareable link is not currently available for this article.
Provided by the Springer Nature SharedIt content-sharing initiative
By submitting a comment you agree to abide by our Terms and Community Guidelines . If you find something abusive or that does not comply with our terms or guidelines please flag it as inappropriate.
Quick links
- Explore articles by subject
- Guide to authors
- Editorial policies
Sign up for the Nature Briefing newsletter — what matters in science, free to your inbox daily.


IMAGES
VIDEO
COMMENTS
Answer. Please check the IPMI event log, and also observe these system behavior when CPU temperature starts to rise. 1) When CPU temperature starts to raise (heavy I/O processing), you will see "Processor Automatically Throttled - Assertion" in the IPMI event log. 2) System/CPU fans start picking up speed to counter the rising temperature.
Solution 2: Check Thermal Paste and Heat Sink. Solution 3: Disable Overclocking. Solution 4: Disable Background Processes. Solution 5: Increase RAM. To avoid data loss due to unexpected errors like processor thermal trip error, you'd better make a Windows backup via PC cloning software like MiniTool Partition Wizard.
If you want to fix the processor thermal trip issue on your Windows 11/10 computer, then you should disable CPU overclocking, clean the CPU fan, and power supply fan, check the thermal paste, etc ...
7 2019/08/02 20:52:46 CPU1 Temp Processor Thermal Trip - Assertion 8 2019/08/02 20:52:46 CPU2 Temp Processor Thermal Trip - Assertion. Answer: Since the CPU shows the same symptom on both motherboards, you will need to get a different CPU to validate if it is the defective CPU that sends out the false sensor readings or not?
Exclude CPLD FW issue, it looks like CPLD intermittently reports the fake event log of Thermal Trip. We suggest to confirm the fan speed and chassis not-opened, clear all the event logs then check if still CPLD events. BTW, did you ever flash IPMI FW and choose preserve configuration ? If yes, we suggest to make a clear FW flash and keep ...
'Thermal Trip error' - Processor Thermal Trip error: ... This issue can be occurred due to insufficient RAM or memory space in computer. You can increase RAM from 2GB to GB in order to fix the issue, and then check if it works for you. Method 6: Check core temperature.
Therefore, it is important to check the thermal paste and heat sink on your processor: 1. Remove the fan by carefully unscrewing the bolts just right above your processor. 2. Apply the cooling agent on top of the processor. 3. Now, screw the fan again in its place. 4.
WARNING : Processor Thermal Trip. Press any key to continue. . . These types of warning messages are always thrown went the CPU of the desktop or laptop's processor gets heated up above its threshold temperature. The reasons why this happened on the Windows system are listed below. Many heavy applications are running simultaneously on any system.
Solution 1] Check the Task Manager for high CPU usage. The fan runs faster and the system puts more resources to use when a certain process uses more of system resources and strains the system. 1] Press CTRL+ALT+DEL to open the Security Options menu. 2] Select Task Manager from the list and expand the window to show all processes.
A. Check the Fans. Fans are the main part of the cooling system. If the fans stop working, CPU and other components will overheat and cause the processor thermal trip. 1. Shut down your system. Make sure that you have the power switch also turned off. 2. Then, carefully open the rear cabinet. 3.
Solution 3: Checking thermal paste and heat sink. If the fans are working alright with ventilating the air and you still get an error, chances are that the thermal paste on your processor is not applied correctly. Thermal paste is a substance which acts as a cooling agent and drastically cools your processor through the fan installed just above ...
342. January 19th, 2024 02:08. Poweredge R430 will not power on. CPU1 Thermal Trip event. The server attempts to power on, the fans rev up for a second then it shuts off. IDRAC displays CPU1 CPU0001 Thermal trip event. check fans and replace heatsink. I have checked and all the fans are fine.
There is no threshold for CPU Thermal-trip shutdown. Regardless of whether Thermal Monitor is enabled, in the event of a catastrophic cooling failure, the processor will automatically shut down when the silicon has reached an elevated temperature. At this point, the THERMTRIP_N signal will go active and stay active.
3. Check thermal paste and heat sink. There is also a less common case where the processor overheats due to insufficient thermal paste between the CPU and its heat sink. Removing the old thermal paste and reinstalling the heat sink will be the only solution. Find a professional repair service if you are unable to perform this operation. 4.
Inadequate thermal grease on the heat sink can cause unusually high processor temperatures. *Make sure your system's I/O (disk, floppy, CD-ROM, etc.) cables aren't restricting airflow in the system. *Please ensure that both the power supply and chassis comply with the ATX 2.03 standard (also called "ATX12V").
Symptoms indicate dead motherboard: - CPU is not powering on. It stays cool. - MB starts and shuts down immediately or starts and hangs there. That keeps on changing. MB is responsive to the power button. - No display no beeps. - Network chip powers on. - SB felt cold but that may be normal.
Resolution. The Processor Thermal Trip event initially means the processor got so hot that its over-temperature protection was triggered and the system was shut down to prevent damage (qtd. on page# 49 of the System Event Log (SEL) Troubleshooting Guide). If this was a one-time event; if the fans are currently working fine and temperature is normal, proceed clearing the System Event Log and ...
The Baseboard Management Controller (BMC) System Event Log (SEL) contains repeated entries for Central Processing Unit (CPU) Thermal Trip events. Affected configurations. The system may be any of the following IBM servers: System x3450, type 7948, any model. This tip is not software specific. This tip is not option specific.
What does thermal trip indicate? Answer: Thermal trip is a CPU internal hardware over heat protection. Once the CPU detects the temperature is over the limitation, it will trigger a system shutdown. It is a pure hardware control in the CPU and depends on which CPU you use. IPMI will only monitor this status, once it is triggered it will logged.
P1D0 - processor 1, die 0. P1D1 - processor 1, die 1. Therefore, the message "Memory Mem P1D1 Th Trip" means Processor 1, Die 1 was overheated and a thermal trip (Th Trip) event occurred. Note. It does not mean the memory DIMM in D1 slot has is defective. Memory (DIMMs) are sensitive to excessive heat and may cause the server to be unstable ...
R820 报错cpu0001 提示 cpu1 has a thermal trip. Hi, 关于这个报错,建议先释放静电试试,具体方法:移除电源线,按着开机按钮大概30秒先释放一下静电,重新插入电源线后 , 请先等待一分钟再接通服务器电源测试。. 假如问题没有解决,留意一下服务器状态灯的情况 ...
If the CPU IERR has been caused by an operating system event the Operating System Event Log should be checked and cross referenced with the Server System Event Log to identify the Operating System event that has caused the CPU IERR. Once this operating system event has been identified, the operating system provider should be contacted to assist ...
For technical support, please send an email to [email protected]. Enter your email address below if you'd like a technical support staff to reply: FAQ Stats. FAQ ID. Related Category / Keyword. Date Posted. Code. 13977. N/A.
The debate surrounding the roles of thermal and non-thermal pathways in plasmonic catalysis has captured the attention of researchers and sparked vibrant discussions within the scientific community.